Detection of microorganisms using cellular component-based rapid method technologies
Posted: 20 June 2011 |
This is the third in a series of articles on rapid microbiological methods that will appear in European Pharmaceutical Review during 2011. In my last article, I provided an overview of viability-based rapid microbiological methods (RMMs), such as flow and solid-phase cytometry. In this article, we will review some of the currently available RMMs that fall under the category of cellular-component based technologies. These RMMs rely on the analysis of cellular markers or the use of probes that are specific for microbial target sites of interest. Examples include ATP bioluminescence, the detection of endotoxin and the use of MALDI-TOF mass spectrometry for microbial identification.
ATP bioluminescence is the generation of light by a biological process, and is most recognised in the tails of the American firefly Photinus pyralis. First discovered in 1947 by William McElroy, he described the ATP bioluminescence reaction in which ATP (Adenosine Triphosphate) is enzymatically consumed to produce light.
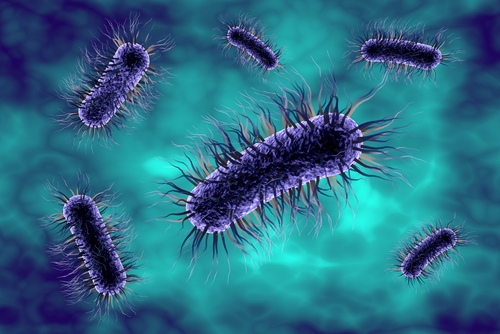
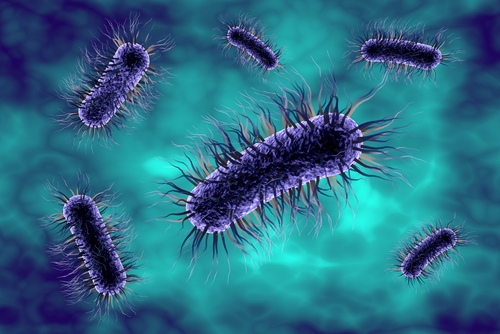
This is the third in a series of articles on rapid microbiological methods that will appear in European Pharmaceutical Review during 2011. In my last article, I provided an overview of viability-based rapid microbiological methods (RMMs), such as flow and solid-phase cytometry. In this article, we will review some of the currently available RMMs that fall under the category of cellular-component based technologies. These RMMs rely on the analysis of cellular markers or the use of probes that are specific for microbial target sites of interest. Examples include ATP bioluminescence, the detection of endotoxin and the use of MALDI-TOF mass spectrometry for microbial identification.
ATP bioluminescence is the generation of light by a biological process, and is most recognised in the tails of the American firefly Photinus pyralis. First discovered in 1947 by William McElroy, he described the ATP bioluminescence reaction in which ATP (Adenosine Triphosphate) is enzymatically consumed to produce light. Specifically, in the presence of the substrate luciferin, the enzyme luciferase will use the energy from ATP to oxidise luciferin and release photons. The photons can then be detected and measured by a luminometer equipped with a photomultiplier tube.
Because all living cells store energy in the form of ATP, this cellular component can be used as a measure of organism viability. Basically, we can capture the microorganisms of interest, release the ATP from within these cells, add the luciferin and luciferase reagents and measure the amount of bioluminescence generated. The sensitivity for ATP bioluminescence (i.e., how many cells are required to detect a sufficient amount of photons or light) is between 100-1000 bacterial cells and a single yeast/mould cell. For this reason, when low numbers of bacterial cells are expected in a test sample, an enrichment step in media may be required to allow these cells to multiply and produce a sufficient level ATP for subsequent detection.
A number of ATP bioluminescence systems are currently in use within our industry. Many will provide data in terms of a relative light unit (RLU), which can be correlated with viable cell concentration. In this regard, these systems are primarily used for estimating the cell count based on the specific amount of light that is detected, or for presence/absence testing. The latter can be accomplished by under – standing what the lower level of detection is (in terms of RLUs), and if the results are below the level of detection, then no viable organisms are present. This is the basis for the manner in which GSK uses ATP bioluminescence for the early release of a non-sterile prescription nasal spray (i.e., up to four days earlier release than conventional methods).
Another RMM utilises a more direct enumeration approach by using a membrane filtration technique that employs a hydrophilic filter containing more than 600 compartments separated by hydrophobic partitions. The test sample is filtered through this membrane and individual cells are captured within these compartments. The filter is then transferred onto a suitable agar medium, and the single cells will form microcolonies during a short incubation period. The filter is subsequently treated with an ATP-releasing agent, and the same luciferin and luciferase reagents as described above, and then a special luminometer is used to intensify the bioluminescence from each microcolony. The bioluminescence signals are then quantified, and a cell count (i.e., the number of micro – colonies arising from a single cell) is provided.
ATP bioluminescence is limited by the fact that an organism can contain only a finite amount of ATP (e.g., an average bacterial cell contains 1 attomole of ATP). For this reason, one RMM supplier developed a novel method to amplify the amount of ATP generated in a cell. All living microorganisms contain adenylate kinase (AK), another vital part of energy metabolism. Because AK is an enzyme, rather than a metabolite, it is possible to use AK to generate almost unlimited amounts of its products, including ATP. For example, AK catalyses the linear amplification of ADP to high levels of ATP. Therefore, if we extract both ATP and AK from a cell, add ADP, luciferin and luciferase, the resulting reaction can produce a 1000-fold increase in ATP, which is detected by the supplier’s luminometer.
Today, many companies have employed the use of ATP bioluminescence for presence / absence testing, bioburden estimations, hygiene monitoring and most recently, finished product sterility testing.
Endotoxin testing
Today, the most widely used methods for endotoxin detection employ Limulus Amebocyte Lysate (LAL), which is isolated from the blood of the horseshoe crab (Limulus polyphemus). Many laboratories utilise bench-top instruments for this type of testing, which require samples to be transferred from the manufacturing floor or other locations to the laboratory. Recent advances in endotoxin instrumentation now allow for testing to be performed at the point-of-use or point-of-sampling, with results in as early as 15 minutes. One such LAL-based technology uses a disposable cartridge and hand-held incubating spectrophotometer to allow for a quantitative, kinetic chromogenic method by measuring the colour intensity directly related to the endotoxin concentration in a test sample. Each cartridge contains LAL reagent, chromogenic substrate and control standard endotoxin. The sensitivity of this RMM is 0.01-1.0 and 0.05-5.0 EU per mL, and is FDA-approved as an alternative to traditional LAL testing methods for final product release. Many companies are now using this technology for testing pharmaceutical grade water systems (at the point-of-use), raw materials, in-process samples and finished products.
Fatty acid analysis
The cellular membrane contains lipid biopolymers, and we can utilise fatty acid profiles to provide a fingerprint for micro – organism identification. One available RMM uses gas chromatography (GC) to analyse fatty acids that have been extracted from microorganisms after a pure culture has been obtained. After a series of relatively simple chemical conversions, methylation reactions and washing steps, the purified fatty acids are analysed in a GC, and the resulting data peaks are compared with an internal database of fatty acid peaks obtained from processing known microorganisms through the system. If a match is found, the microbial identity of the microorganism is provided.
MALDI-TOF Mass Spectrometry
Matrix Assisted Laser Desorption Ionisation – Time of Flight (MALDI-TOF) mass spectrometry can provide an accurate molecular weight measurement and characterisation of biomolecules, including proteins, peptides, polysaccharides and nucleic acids. Therefore, it is possible to use MALDI-TOF to generate a mass spectrum from microorganisms that can be used for microbial identification. Basically, a biomolecule (or intact microbial cell) is combined with a UV-absorbing matrix on a stainless steel plate (usually the size of a small microtitre plate), and then a laser ionises the resulting mixture. The matrix absorbs the energy from the laser, preventing unwanted fragmentation of the biomolecule. The ionised particles are then accelerated in an electric field and enter what is known as the flight tube. Within the flight tube, different molecules are separated according to their mass to charge ratio, and as a result, they reach the mass spectrometry detector at different times (e.g. lighter ions will reach the detector faster than heavier ions). Within a few seconds, a mass spectrum is generated, and this spectrum is then compared with an internal database containing similar spectra from known microorganisms. MALDI-TOF RMMs are now available for this purpose, and the user will normally use a pure culture as the starting material (~ 105 viable cells are required for an accurate measurement). A portion of an isolated colony is smeared onto the target plate and allowed to co-crystallise with the UV-absorbing matrix. After drying, the target plate is placed into the mass spectrometer, and exposed to the laser. Ionised proteins and peptides are arranged in a spectrum with increasing mass, and the resulting mass spectra are compared with the internal database.
SELDI-TOF Mass Spectrometry
Surface Enhanced Laser Desorption Ionisation – Time of Flight (SELDI-TOF) mass spectrometry is similar to MALDI-TOF, except that the system currently in use employs a chip array technology that contains either a chemical (e.g., ionic, hydrophobic, hydrophilic) or biochemical (e.g., antibody, receptor, DNA) surface designed to capture specific proteins of interest. This enables direct profiling of proteins from complex biological samples, bypassing the complicated steps of purification. MALDI-TOF MS of the bound protein molecules is subsequently performed. This type of RMM is routinely used for protein profiling in drug discovery and disease diagnostics; however, we may see similar systems introduced for routine microbial identification in the future.
Fourier Transform-Infrared (FT-IR) Spectrometry
Another cellular-component based RMM utilises Fourier transform-infrared spectrometry, or FT-IR. This type of spectrometry has been successfully used in pharmaceutical manufacturing and quality control to provide positive fingerprint identification of materials. Each molecule’s functional group within a particular material under analysis can absorb infrared radiation to generate a characteristic absorption or transmission spectrum. FT-IR can also generate spectra from microorganisms without the need for extraction, amplification, labelling or staining of any kind. Cellular material from a pure culture is spread onto a microplate and dried at 40 – 45°C under vacuum to create a biofilm. The dried biofilm is then analysed in the FT-IR spectrometer. Each cell’s FT-IR spectra reflects its biochemical composition, including proteins, lipids, DNA and RNA, and carbo – hydrates. This spectral fingerprint can then be compared with other spectra within a previously generated database, and if a match if found, an identification is provided.
Summary
Cellular component-based rapid micro – biological methods have been used for a wide range of applications, including the detection, enumeration and identification of microorganisms. Furthermore, a number of different technologies are currently available that are based on a variety of distinctive scientific principles. It is up to the end-user to determine the right technology for the intended application, as each system can differ in their level of detection, specificity, throughput, compatibility with sample types, ease of use, and cost. I discuss many of these validation and implementation considerations on my website, http://rapidmicromethods.com, and I encourage you to visit for additional infor mation and guidance.
Join me for the next article in this series, where we will discuss the use of other types of spectroscopic-based RMMs, such as light scattering technologies, for microbial detection, identification and real-time environmental monitoring.
About the Author
Dr. Michael J. Miller is an internationally recognised microbiologist and subject matter expert in pharmaceutical microbiology and the design, validation and implementation of rapid microbiological methods. He is currently the President of Microbiology Consultants, LLC (http://microbiologyconsultants.com). For more than 22 years, he has held numerous R&D, manufacturing, quality, and consulting and business development leadership roles at Johnson & Johnson, Eli Lilly and Company, Bausch & Lomb, and Pharmaceutical Systems, Inc. In his current role, Dr. Miller consults with multinational companies in providing technical, quality and regulatory solutions for pharmaceutical manufacturing, contamination control and microbiological PAT. He also provides comprehensive training for his clients in the areas of rapid method validation and implementation.
Dr. Miller has authored over 100 technical publications and presentations in the areas of rapid microbiological methods, PAT, ophthalmics, disinfection and sterilisation, is the editor of PDA’s Encyclopedia of Rapid Microbiological Methods, and is the owner of http://rapidmicromethods.com, a website dedicated to the advancement of rapid methods. He currently serves on a number of PDA’s program and publication committees and advisory boards, is co-chairing the revision of PDA Technical Report #33: Evaluation, Validation and Implementation of New Microbiological Testing Methods, and routinely provides RMM training programs for the industry and professional organisations worldwide.
Dr. Miller holds a Ph.D. in Microbiology and Biochemistry from Georgia State University (GSU), a B.A. in Anthropology and Sociology from Hobart College, and is currently an adjunct professor at GSU. He was appointed the John Henry Hobart Fellow in Residence for Ethics and Social Justice, awarded PDA’s Distinguished Service Award and was named Microbiologist of the Year by the Institute of Validation Technology (IVT).