Next generation antibody therapeutics: Antibody fragments, dual-targeting strategies, and beyond…
Posted: 9 October 2009 | | No comments yet
A multitude of novel therapeutic antibody formats based on modification of the conventional IgG format have arisen in recent years. The intensification of interest in this area reflects a pressing need for an additional repertoire of therapeutic molecules which retain the exquisite binding specificity and low intrinsic toxicity of monoclonal antibodies (mAbs), while engineering additional features which enhance clinical efficacy, for example, by improving tissue perfusion or demonstrating increased specificity for a defined cell type. This article briefly reviews the key molecular aspects of therapeutic antibody fragments in development, including single-chain Fv fragments (scFvs), camelid VHH domains, human domain antibodies (dAbs), and an expanding array of bispecific/dual-targeting variants. Such molecules, characterised by unique biophysical and pharmacological properties, are ideally placed to become the next generation of antibody-based therapeutics.
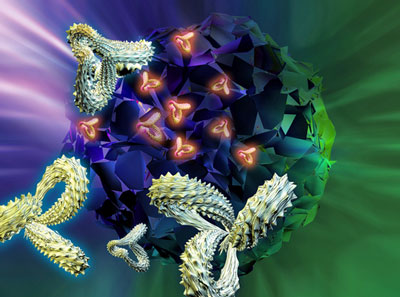
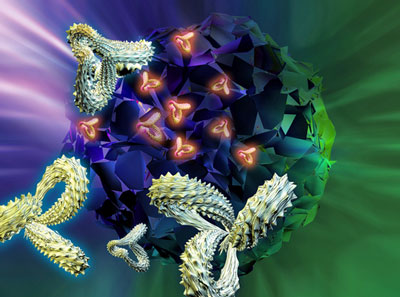
A (very) brief history of antibody therapeutics…
The successful use of antibodies as a therapeutic modality began at the end of the 19th century with the pioneering work of Emil von Behring and Shibasaburo Kitasato1, as highlighted in Figure 1. However, it was not until the mid-1970’s when César Milstein and Georges Köhler established a method to prepare reproducibly continuous cultures of monoclonal antibodies (mAbs) from murine B lymphocytes2, that a powerful research and diagnostic tool with the potential to transform medicine was revealed. In the early 1980s several attempts were made to exploit this novel technology as a therapeutic strategy – however, largely due to the inherent immunogenicity of mouse mAbs, most failed during clinical evaluation3. The advent of robust molecular biology techniques was fundamental in overcoming these issues – cloning of immunoglobulin genes and the subsequent development of human-mouse chimaeric antibodies4 effectively removed the most immunogenic portion of a mouse mAb with a concomitant reduction in the anti-drug response (human anti-mouse antibody response, HAMA). Closely following the development of human-mouse chimaeras, Greg Winter’s group at the MRC Laboratory of Molecular Biology (Cambridge, UK) demonstrated grafting of the complimentarity-determining regions (CDRs) from mouse-derived monoclonals into a human variable region framework5, thereby successfully ‘humanising’ mouse mAbs (see Figure 1). The production of recombinant antibodies derived entirely from the human immunoglobulin repertoire (termed ‘fully human’) was made possible by the development of transgenic mice incorporating human germline sequences, or through the use of phage display – a process whereby bacteriophage are engineered to express extensive repertoires of antibody ‘libraries’ which can be propagated and selected to permit identification of particular antibodies or fragments (see Figure 1)6,7. By the mid-1990’s a series of FDA-approved antibody therapeutics had reached the wider patient population, comprising chimaeric mouse/human mAbs and humanised antibodies, closely followed by the first fully human mAbs.
Key characteristics and limitations of therapeutic antibodies
Several features of the conventional IgG format have sustained interest in this therapeutic modality8, as shown in Figure 2. First of all, antibodies show exquisite binding specificity, thereby limiting off-target effects and improving the clinical safety profile. Secondly, humanised and fully human therapeutic antibodies show low intrinsic toxicity and immunogenicity since very similar molecules are naturally present at significant concentrations in the circulatory system. Another key characteristic of the IgG format is the inherent ability to recruit immune cell function to the site of antigen expression via the fragment crystallisable (Fc) – these effector functions may be tailored to suit the therapeutic target and desired pharmacological properties by modification of the Fc region. Furthermore, such molecules can be adapted to specifically direct a payload of drug (radionuclide, bacterial toxin, or plant-derived toxin such as ricin) or pro-drug to a particular cell type or tissue, thereby reducing systemic toxicity associated with such molecules. Finally, efficient recycling of IgG sub-types 1, 2 and 4 by the neonatal Fc receptor (FcRn) significantly prolongs serum half-life of such molecules, thereby reducing the dosing interval required to elicit a therapeutic effect.
The limitations of traditional antibody-based therapies (also highlighted in Figure 2) include the high ‘cost of goods’ associated with production of complex biomolecules in mammalian cell culture systems9. In addition, the size of the molecule, coupled with sensitivity to proteolytic degradation and the quantities of clinical grade material required to obtain a therapeutic effect, limits delivery routes to intravenous and subcutaneous delivery, and in so doing reduces the range of therapeutic indications that would warrant such approaches. Importantly, tissue perfusion is limited with full-length mAbs due to their large size, which may reduce efficacy in the treatment of solid tumours10-12; in addition, the large size of mAbs could also impede binding to epitopes that are potentially accessible to smaller molecules13. A key characteristic of the IgG format is that the molecule is bivalent but monospecific – in other words, antibodies are typically able to bind two molecules of a single target. However, in many clinical applications it may be advantageous to neutralise two or more soluble targets, or to enhance specificity of the molecule by binding two separate cell surface molecules in order to ensure destruction of a defined cell type or tumour without affecting the function or viability of surrounding cell types. For example, blockade of two overlapping growth factor-mediated pathways or cytokine-induced cellular signalling pathways may exhibit a synergistic clinical effect14; in addition, homing of antibodies to particular cell types may be significantly improved by targeting two cell surface receptors to selectively bind to cells that co-express both antigens15.
Recently, a range of novel antibody formats have been described which are predicted to offer advantages over the current IgG format either by improving clinical efficacy via neutralisation of more than one target antigen, allowing manufacture in alternative (usually prokaryotic) expression system, or increasing perfusion of the molecules and/or permitting an alternative delivery route to the desired site of action as a result of smaller size and improved biophysical properties. Currently, such novel antibody formats have yet to reach late stage clinical trials but due to the benefits demonstrated in preclinical in vivo models and early clinical trials, efforts to investigate the potential of such therapeutic approaches are expected to intensify. The wide range of therapeutic antibody fragments and dual targeting molecules currently under evaluation is presented in the following sections.
Therapeutic antibody fragments
Although the market is currently dominated by antibodies derived from the conventional IgG format, three therapeutic Fab fragments are already approved by the FDA – these are ReoPro® (abciximab, an anti-gpIIb/IIIa Fab fragment for prevention of blot clots in angioplasty), Lucentis® (ranibizumab, an anti-VEGF Fab fragment indicated for the treatment of the wet form of age-related macular degeneration), and Cimzia® (certolizumab pegol, a PEGylated anti-TNFα Fab fragment for Crohn’s disease and rheumatoid arthritis)16. These Fab fragments represent the simplest form of antibody-derived molecules formed by proteolytic digestion or by deletion of the Fc coding sequence (see Figure 3a). However, advances in antibody engineering and expanding knowledge of the structure and function of IgG subclasses continues apace, and a diverse array of next generation antibody fragments have entered pre-clinical testing and clinical trials.
Single-chain Fv fragments (scFvs), in which the heavy and light chain variable domains (VH and VL) are paired using a flexible linker that stabilises the interaction between the two domains, represent further truncation of Fab fragments through deletion of the CH1 and CL domains (see Figure 3a)18-20. Despite mutual stabilisation of the domains offered by the linker, most scFvs show poor to moderate stability18 – however, stability and folding of variable domains may be significantly improved by point mutations or CDR grafting to more stable VH domain frameworks21. Indeed, a scFv fragment (Pexelizumab, anti-complement C5) was sufficiently stable and highly expressed to progress to Phase III clinical trials22, although ultimately failing to meet its primary endpoint in myocardial infarction; interestingly, this scFv fragment was shown to be efficiently aerosolised supporting investigation as a potential treatment for respiratory diseases such as severe acute allergic asthma23,24.
Interest in antibody fragments as a therapeutic modality was recently revived by the discovery that high affinity and stable domains linked to the equivalent Fc region have evolved in camelids (camels and llamas) and sharks (see Figure 3a)25-28. Nanobodies® (Ablynx) represent the commercialisation of these studies; such molecules lacking the Fc-equivalent region are termed VHH domains, representing a single camelid heavy chain variable domain (see Figure 3a). As for many antibody fragments, a key advantage of VHH antibodies is the ability for high-level expression in prokaryotic systems, enabling ease of production and reduced cost of goods29. Furthermore, camelid VHH domains display long surface loops which reportedly enable improved penetration and contact with normally inaccessible epitopes, such as enzyme active sites and microbial antigens which have evolved to evade detection by conventional IgG molecules30. Two distinct VHH antibody fragments targeting von Willebrand Factor (vWF) are currently progressing through clinical trials as novel anti-thrombotic strategies; additional molecules are in development for osteoporosis (targeting RANKL) and for multiple inflammatory disorders (targeting TNFα)31.
Although VHH domains represent single domain antibody-based therapeutics, the smallest known antigen-binding fragments derived from human antibody repertoires are known as domain antibodiesTM (dAbsTM; GlaxoSmithKline) (See Figure 3a). Such molecules range in size from 11 to 15 kDa (compared with ~57 kDa for recombinant Fab fragments and ~27 kDa for scFvs), and represent the single variable regions of the human heavy (VH) or light chain (VL). Since their initial discovery, advances in selection and expression have revealed dAbs to be a robust and versatile platform for therapeutic intervention32. The biophysical properties of dAbs have been significantly improved using methods including site-directed mutagenesis and in silico molecular modelling33; similarly, the in vitro maturation of dAbs and other recombinant antibody fragments has been greatly improved by methods including error-prone PCR and bacterial mutator strains34,35, in addition to more targeted techniques such as CDR shuffling36. As for VHH antibodies, dAbs are relatively small, unglycosylated proteins and can therefore be produced at high levels in a functional form in prokaryotic expression systems, representing a potentially more straightforward and economical route to large-scale production. The first dAb-based therapeutics are currently progressing through pre-clinical testing and into early-stage clinical trials, including those for treatment of COPD and an anti-TNFα molecule for inflammatory disorders37.
In many therapeutic applications a potential disadvantage of domain antibody formats and other small antibody fragments is a short half-life since molecular weights are often below the cut-off for renal clearance38. However, this may be circumvented by the addition of polyethylene glycol (PEGylation) or addition of an Fc region to the molecule (see Figure 3a)39,40; the latter retains some of the advantages of small size while improving half-life via FcRn-mediated recycling, in addition to restoring the ability to manipulate effector functions. A further strategy to improve serum half-life involves fusion of the antibody fragment to an additional domain which binds serum albumin – such molecules, termed AlbudAbsTM (GlaxoSmithKline), have demonstrated dramatically improved in vivo efficacy due to the extension in serum half-life41. Intriguingly, serum albumin is known to accumulate in tumours and in arthritic joints which may suggest a further degree of targeting to such tissues, thereby further enhancing clinical efficacy41; such antibody fragments would also be expected to enhance perfusion into poorly vascularised tumours and other tissues10-12.
A major goal of research into novel antibody fragments is the ‘Holy Grail’ of oral and/or pulmonary delivery of antibody-derived therapeutics – thereby circumventing the need for intravenous infusion or subcutaneous injection. Of these approaches, delivery to the respiratory tract and deep lung is the most promising since the physiological barriers for uptake are lower than oral delivery, although there are some concerns about the long-term effects of inhaling protein therapeutics which need to be addressed42. Due to optimised biophysical properties, single-domain antibodies may be more resistant to heat and pH thereby retaining their activity as they pass through the gastrointestinal tract and might also be of a sufficiently small size to penetrate the deep lung. Such properties are raising the hope of pulmonary delivery for respiratory conditions, and indeed any disease that may respond favourably to a systemically-delivered antigen-binding domain directed against clinically relevant targets.
Dual targeting formats
Dual targeting, or bispecific, antibodies are single molecules that comprise at least two distinct antigen-binding specificities. Dual targeting antibodies therefore represent a distinct class of molecules which offer the promise of developing completely novel therapeutic strategies with advantages over the conventional IgG format. A simplistic approach to producing such molecules involves chemical cross-linking of two antibody fragments43,44, although preparation of such molecules is often laborious and relatively expensive8. It has long been known that fusion of two hybridoma cell lines creates ‘quadromas’45,46 – however due to the random assortment of the heavy and light chains, a mixture of multiple discrete antibody structures are produced, of which only one has the desired binding specificity. Interestingly, a technique has been devised to reduce this heterogeneity resulting in the first approval in the EU for a dual-targeting approach for the treatment of malignant ascites in patients positive for a key tumour antigen47,48. Nevertheless, it has been necessary to devise strategies to create dual targeting molecules in a more defined manner. The major types of dual targeting strategies that are emerging from these current efforts are discussed below and shown schematically in Figure 3b.
Initial attempts to engineer dual targeting molecules sought to overcome the major limitations of chemical cross-linking by the addition of a protein linker to create bispecific/tandem scFvs (see Figure 3b) – however, such molecules are often poorly soluble and may need to be refolded from inclusion bodies or expressed in mammalian cells49-51. An alternative approach to generating bispecific scFvs, termed ‘diabodies’ (Dbs), involves shortening the linker between the VH and VL domains such that the domains cannot assemble, thereby forcing them to pair with the VH and VL domains from another polypeptide (see Figure 3b)52. This format is desirable from a manufacturing perspective as diabodies can be expressed at high levels in bacteria or yeast53.
Additional development of the scFv format has led to the generation of single molecular entities capable of binding three or more distinct antigens17. The most successful approach to engineering multimeric scFvs appears to be further reduction of the domain linker length thereby driving formation of trivalent trimers (triabodies, 80 kDa) (see Figure 3b)54, or tetravalent tetramers (110 kDa)55. Notably, such multivalent formats should retain the potential for improved tumour penetration due to their small size relative to the conventional IgG format; the multimeric nature of such formats would also be expected to significantly increase functional affinity (avidity) of the molecule for a single target56.
An area of increasing interest is the recruitment of T-lymphocytes by an array of different dual targeting platforms, ultimately aiming to enhance lysis of a selected target cell type – such a strategy is necessary since conventional IgG molecules are unable to directly engage T cells due to lack of Fcγ receptors on the cell surface. One example of a dual targeting strategy capable of directing cytotoxic T cells to target cells is termed ‘BiTE’ (Bispecific T-cell-engager), a bispecific scFv format specifically engineered to engage cytotoxic T cells for lysis of bound tumour cells. These molecules are designed to bind simultaneously to the CD3 complex present on every T-cell population and to a selected cell-surface tumour antigen – since binding to CD3 occurs with low affinity it only triggers key signalling events if the bound molecule is presented to the T-cell in a multivalent manner by a labelled target cell57. This mode of action therefore connects cytotoxic T cells to potentially any cancer cell or tumour type, independent of T-cell receptor specificity, co-stimulatory molecules, or antigen-presentation complexes. This format is highly effective in in vitro cell-based assays and in vivo models and has been demonstrated to trigger lysis at very low effector:target ratios57. Two BiTE molecules, using either CD19 or Ep-CAM as the tumour-targeting moiety, are currently being tested in clinical trials for non-Hodgkin’s Lymphoma58, B-precursor acute lymphoblastic leukaemia, and colorectal cancer.
The small size of dAbs and camelid VHH domains has opened up unique opportunities for multimerisation; such domains may be fused using a short linker sequence allowing neutralisation of two or more related or distinct molecular targets59. Such fusions are not possible with conventional IgG molecules and difficult to achieve with Fabs and scFvs, not least because pairing of the domains is not required for stabilisation of the molecule. A common linker used to fuse together two domain antibodies is a modified version of the flexible IgG hinge region60, designed to ensure independent movement of the coupled variable domains and also to reduce the potential for immunogenicity. The domain antibody in-line fusion format is exemplified by the AlbudAb platform designed to enhance the serum half-life of domain antibodies41 but could be further exploited to bind a wide range of different target antigens.
An array of additional bispecific/dual targeting antibody formats represent subtle modification of the full-length IgG format – these include an intriguing approach involving selection of antibodies capable of binding two distinct antigens61. In the reported example, single antibody molecules were selected to bind two unrelated antigens (ErbB3/Her2 and VEGF) with high affinity on both ‘arms’ of the molecule, thereby suggesting a new paradigm in our understanding of the properties of antibodies and also in the development of dual targeting strategies. An alternative approach involves fusion of antibody fragments to full-length IgG to create bispecific molecules – examples of this approach include the dual-variable domain Ig (DVD-IgTM, Abbott) and mAb-ScFv formats (Biogen Idec; MedImmune), which both represent fusion of a defined antibody variable domain or ScFv to another full-length IgG molecule to allow neutralisation of two antigens in one molecule (see Figure 3B). In both cases, the formats appear to allow production of highly stable molecules with IgG-like pharmacokinetic properties62,63.
Conclusions
While initial interest in monoclonal antibodies as therapeutic agents has taken some time to translate into genuine success in the clinic3, such molecules are gaining renewed attention from patients, clinicians, and the pharmaceutical industry alike due to high profile successes in the clinic64. It is now estimated that a significant proportion of new drugs expected to be licensed during the next decade will be based on antibody products64-67 and an array of novel formats are being evaluated to extend the clinical applications of conventional IgG molecules8. The development of such novel molecular scaffolds, broadly comprising engineered antibody fragments and dual targeting frameworks, has unlocked entirely new therapeutic perspectives. Further development of such molecules in a rational manner, aiming to combine robust pharmacokinetic profiles with efficient methods of large-scale production, and unambiguous demonstration of synergistic clinical efficacies using dual targeting/bispecific variants, will be critical to the continued success of these novel antibody formats.
Acknowledgements
We would like to thank Paul Hamblin and Martin Orecchia for critical reading of the manuscript.
ReoPro is a registered trademark of Eli Lilly and Company; Lucentis is a registered trademark of Novartis AG; Cimzia is a registered trademark of UCB Inc.; Orthoclone is a registered trademark of Biogen Idec; Rituxan, Herceptin and Zenapax are registered trademarks of F. Hoffman-La Roche Ltd.; Humira is a registered trademark of AstraZeneca Ltd.; Zevalin is a registered trademark of Bayer AG.
The Nanobody platform is a registered trademark of Ablynx; the Domain antibody and AlbudAb platforms are trademarks of GlaxoSmithKline plc.; the DVD-Ig format is a trademark of Abbott Laboratories Ltd.
References
- Behring E. & Kitasato S. (1890). Über das Zustandekommen der Diphterie-Immunität und der Tetanus-Immunität bei Thieren. Dt Med Wschr 16 1113-1114
- Kohler G. & Milstein C. (1975). Continuous cultures of fused cells secreting antibody of predefined specificity. Nature 256 495-497
- Dübel S. (2007). Therapeutic Antibodies – From Past to Future. In: Handbook of Therapeutic Antibodies, edited by Stefan Dübel, Wiley-VCH & Co., Weinheim
- Neuberger M. et al. (1984). Recombinant antibodies possessing novel effector functions. Nature 312 604-608
- Riechmann L. et al. (1988). Reshaping human antibodies for therapy. Nature 332 323-327
- Hust M et al. (2007). Selection Strategies II: Antibody Phage Display. In: Handbook of Therapeutic Antibodies, edited by Stefan Dübel, Wiley-VCH & Co., Weinheim
- Brüggemann M. et al. (2007). Selection Strategies III: Transgenic Mice. In: Handbook of Therapeutic Antibodies, edited by Stefan Dübel, Wiley-VCH & Co., Weinheim
- Fischer N. & Léger O. (2007). Bispecific Antibodies: Molecules That Enable Novel Therapeutic Strategies. Pathobiology 74 3-14
- Farid S. (2007). Process economics of industrial monoclonal antibody manufacture. J. Chrom. B. 848 8-18
- Jain R. (1990). Physiological Barriers to Delivery of Monoclonal Antibodies and Other Macromolecules in Tumors. Cancer Res 50 (Suppl.), 814s-819s
- Yokota T. et al. (1992). Rapid Tumor Penetration of a Single-Chain Fv and Comparison with Other Immunoglobulin Forms. J Cancer Res 52 3402-3408
- Ho R. & Gibaldi M. (2003). Antibodies and Derivatives. In: Biotechnology and Biopharmaceuticals, by Rodney Ho and Milo Gibaldi. John Wiley & Sons, Inc., New Jersey
- Ward E. et al. (1989). Binding activities of a repertoire of single immunoglobulin variable domains secreted from Escherichia coli. Nature 341 544-546
- Lu D. et al. (2005). A fully human recombinant IgG-like bispecific antibody to both the epidermal growth factor receptor and the insulin-like growth factor receptor for enhanced antitumor activity. J Biol Chem 280 19665-19672
- Robinson M. et al. (2008). Targeting ErbB2 and ErbB3 with a Bispecific-Single Chain Fv Enhances Targeting Selectivity and Induces a Therapeutic Effect in vitro. Br J Cancer 99 1415-1425
- Nelson A. & Reichert J. (2009). Development Trends for Therapeutic Antibody Fragments. Nat Biotech 27 331-337
- Holliger P. & Hudson P. (2007). Engineered antibody fragments and the rise of single domains. Nat Biotech 23 1126-1136
- Bird R. et al. (1998). Single-chain antigen-binding proteins. Science 242 423-426
- Huston J. et al. (1991). Protein engineering of single-chain Fv analogs and fusion proteins. Methods Enzymol 203 46-88
- Huston J. et al. (1993). Medical applications of single-chain antibodies. Int Rev Immunol 10 195-217
- Honegger A. (2007). Engineering Antibodies for Stability and Efficient Folding. In: Therapeutic Antibodies, edited by Yuti Chernajovsky and Ahuva Nissim. Springer, Berlin
- Testa L. et al. (2008). Pexelizumab in ischemic heart disease: A systematic review and meta-analysis on 15,196 patients. J Thorac Cardiovasc Surg 136 884-893
- Peng T. et al. (2005). Role of C5 in the development of airway inflammation, airway hyperresponsiveness, and ongoing airway response J Clin Invest 115 1590-1600
- Alexion press release: www.ir.alexionpharm.com
- De Genst E. et al. (2004). Chemical basis for the affinity maturation of a camel single domain antibody. J Biol Chem 279 53593-53601
- Dooley H. & Flajnik M. (2005). Shark immunity bites back: affinity maturation and memory response in the nurse shark, Ginglymostoma cirratum. Eur J Immunol 35 936-945
- Streltsov V. & Nuttall S. (2005). Do sharks have a new antibody lineage? Immunol Lett 97 159-160
- Streltsov V. et al. (2004). Structural evidence for evolution of shark Ig new antigen receptor variable domain antibodies from a cell-surface receptor. Proc Natl Acad Sci USA 101 12444-12449
- Harmsen M. & De Haard H. (2007). Properties, production, and applications of camelid single-domain antibody fragments. Appl Microbiol Biotechnol 77 13-22
- Nuttall S. et al. (2004). Selection and affinity maturation of IgNAR variable domains targeting Plasmodium falciparum AMA1. Proteins 55 187-197
- Ablynx press release: www.ablynx.com
- Holt L. et al. (2003). Domain antibodies: proteins for therapy. Trends in Biotechnology 21 484-490
- Ewert S. et al. (2003). Structure-based improvement of the biophysical properties of immunoglobulin VH domains with a generalizable approach. Biochemistry 42 1517-1528
- Hawkins R. et al. (1992). Selection of phage antibodies by binding affinity. Mimicking affinity maturation. J Mol Biol 226 889-896
- Low N. et al. (1996). Mimicking somatic hypermutation: affinity maturation of antibodies displayed on bacteriophage using a bacterial mutator strain. J Mol Biol 260 359-368
- Marks J. et al. (1992). By-passing immunization: building high affinity human antibodies by chain shuffling. Biotechnology 10 779-783
- Arana Therapeutics (formerly Peptech) press releases: www.arana.com
- Fagerholm U. (2007) Prediction of human pharmacokinetics – renal metabolic and excretion clearance. J Pharm Pharmacol 59 1463-1471
- Chapman A. (2002). PEGylated antibodies and antibody fragments for improved therapy: a review. Adv Drug Deliv Rev 54 531-545
- Powers D. et al. (2001). Expression of single-chain Fv-Fc fusions in Pichia pastoris. J Immunol Methods 251 123-135
- Holt L. et al. (2008). Anti-serum albumin domain antibodies for extending the half-lives of short lived drugs. Prot Eng Des Select 21 283-288
- Siekmeier R. & Scheuch G. (2008). Inhaled Insulin – Does it Become Reality? J Physiol Pharm 59 Suppl 6, 81-11
- Glennie M. et al. (1987). Preparation and performance of bispecific F(ab’ gamma)2 antibody containing thioether-linked Fab’ gamma fragments. J Immunol 139 2367-2375
- Shalaby M. et al. (1992). Development of humanized bispecific antibodies reactive with cytotoxic lymphocytes and tumor cells overexpressing the HER2 protooncogene. J Exp Med 175 217-225
- Cotton R. & Milstein C. (1973). Fusion of two immunoglobulin-producing myeloma cells. Nature 244 42-43
- Suresh M. et al. (1986). Bispecific monoclonal antibodies from hybrid hybridomas. Methods Enzymol 121 210-228
- Trion press release: www.trionpharma.de
- Schmitt M. et al. (2004). Opsonization with a trifunctional bispecific (anti-CD3 x anti-EpCAM) antibody results in efficient lysis in vitro and in vivo of EpCAM positive tumor cells by cytotoxic T lymphocytes. Int J Oncol 25 841-848
- Kipriyanov S. (2003). Generation and characterization of bispecific tandem diabodies for tumor therapy. Methods Mol Biol 207 323-333
- Korn T. et al. (2004). Recombinant bispecific antibodies for the targeting of adenoviruses to CEA-expressing tumour cells: a comparative analysis of bacterially expressed single chain diabody and tandem scFv. J Gene Med 6 642-651
- McCall A. et al. (1999). Isolation and characterization of an anti-CD16 singlechain Fv fragment and construction of an anti-HER2/neu/anti-CD16 bispecific scFv that triggers CD16-dependent tumor cytolysis. Mol Immunol 36 433-445
- Holliger P. et al. (1993). ‘Diabodies’: small bivalent and bispecific antibody fragments. Proc Natl Acad Sci USA 90 6444-6448
- Zhu Z. et al. (1996). High level secretion of a humanized bispecific diabody from Escherichia coli. Biotechnology 14 192-196
- Iliades P. et al. (1997). Triabodies: single chain Fv fragments without a linker form trivalent trimers. FEBS Lett 409 437-441
- Power B. et al. (2003). Generation of recombinant multimeric antibody fragments for tumor diagnosis and therapy. Methods Mol Biol 207 335-350
- Kortt A. (2001). Dimeric and trimeric antibodies: high avidity scFvs for cancer targeting. Biomol Eng 18 95-108
- Baeuerle P. & Reinhardt C. (2009). Bispecific T-Cell Engaging Antibodies for Cancer Therapy. Cancer Res 69 4941-4944
- Bargou R. et al. (2008). Tumor regression in cancer patients by very low doses of a T cell-engaging antibody. Science 321 974-977
- Conrath K. et al. (2001). Camel single-domain antibodies as modular building units in bispecific and bivalent antibody constructs. J Biol Chem 276 7346-7350
- Roux K. (1997). Flexibility of human IgG subclasses. J Immunol 159 3372-3382
- Bostrom J. et al. (2009). Variants of the Antibody Herceptin That Interact with HER2 and VEGF at the Antigen Binding Site. Science 323 1610-1614
- Wu C. (2007). Simultaneous targeting of multiple disease mediators by a dual-variable-domain immunoglobulin. Nat Biotechnol 11 1290-1297
- Michaelson J. et al. (2009). Anti-tumor activity of stability-engineered IgG-like Bispecific antibodies targeting TRAIL-R2 and LTβR. mAbs 1 128-141
- Reichert J. et al. (2005). Monoclonal antibody successes in the clinic. Nat Biotechnol 23 1073 -1078
- Carter P. (2006). Potent antibody therapeutics by design. Nat Rev Immunol 6 343-357
- Moutel S. & Perez F. (2008). Meeting report: “Antibodies-Europe. Engineering the Next Generation of Antibodies”. Biotechnol J 3 298-300
- Reichert J. & Valge-Archer V. (2007). Development trends for monoclonal antibody cancer therapeutics. Nat Rev Drug Discov 6 349-356