PCR and personalised cancer medicine
Posted: 16 December 2010 |
The delivery of personalised medicine is a key goal of modern cancer medicine and refers to the tailoring of anticancer therapy to the molecular characteristics of an individual tumour. To facilitate personalised medicine, it is important to have robust and reproducible means of gaining molecular information about a patient’s cancer that can be used to guide clinical decision-making. There have therefore been tremendous efforts to identify molecular signatures – biomarkers – that can be used to help predict a cancer patient’s prognosis or their likelihood of a response to targeted drug therapies.
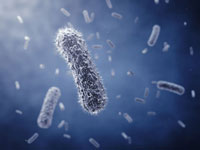
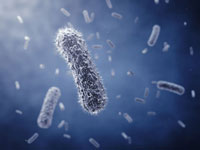
Such molecular profiling has long been applied to haematological malignancies and is increasingly becoming the norm in the most common epithelial cancers such as lung and colorectal cancer. This article will focus on the role of the polymerase chain reaction (PCR) in helping to meet the challenges involved in the design, testing and delivery of personalised cancer medicine.
PCR was first described in 1983 and it is no understatement to say that it has transformed molecular biology. It harnesses the ability of DNA polymerases and flanking oligonucleotide primers to efficiently and precisely replicate a targeted stretch of DNA during each cycle, so that the number of copies of a sequence of interest increases exponentially, thus providing enough material for downstream analysis. With modern thermocyclers, this can be achieved quickly and with minimal hands-on time. Countless modifications of the basic PCR strategy and countless PCR applications have been published. In this article, I will use pertinent examples to illustrate the potential of PCR-based applications in personalised cancer medicine.
Cancer cells are characterised by the acquisition of multiple somatic mutations representing deviations from the ‘germline’ or inherited genome1. The cancer genome therefore is a mixture of inherited ‘normal’ DNA and mutated DNA. Somatic mutations can be alterations in sequence (base substitutions, deletions, insertions), in the number of copies of a section of the genome (deletions, duplications, amplifications – grouped together as somatic CNVs) and translocations, where two parts of the genome are rearranged from their native positions. Genome aberrations often coincide so that, for example, a mutated gene may be selectively amplified2. It is important to note that the cancer genome should be regarded as a dynamically evolving entity. Although it is likely that cancers develop from a single cell, it is becoming increasingly clear that many are also heterogeneous with multiple clonal subpopulations3.
Cancers are usually diagnosed on the basis of abnormal cellular morphology, following long-established histological or cytological techniques. Molecular techniques, including the analysis of nucleic acids can provide complementary information on the presence or absence of somatic mutations that will inform patient stratification to specific biological therapies. The most appropriate technique for mutation detection will depend on the specific genetic loci being interrogated but currently is usually limited to whether or not a mutation in one or two key genes is present. In this article, I will use examples from lung adenocarcinoma (ADC) as illustrations, but the observations can be generalised to all solid tumours. ADC is the most common global cancer. Internationally, mutations in epidermal growth factor receptor (EGFR) and KRAS are often screened for in ADC4. In the UK, it has recently been mandated that all patients with ADC have mutational screening to guide stratification to tyrosine kinase inhibitors (TKI) such as erlotinib or gefitinib with specific activity against mutant EGFR5.
So how are these mutations currently screened for? Direct sequencing (dideoxy – nucleotide) of PCR-amplified DNA was previously considered the gold standard. However, there are now a number of proven methodologies that may have distinct advantages in terms of sensitivity, throughput and analysis. These include pyrosequencing, probe-based detection strategies often using real-time PCR, matrix-assisted laser desorption/ionisation time-of-flight (MALDI-TOF) mass spectroscopy, single strand conformational analysis, denaturing HPLC and high-resolution melting-curve analysis. All of these protocols incorporate a PCR step and in each case the role of PCR is effectively the same – it generates millions of copies of a target DNA sequence in order to facilitate the downstream detection of mutational events.
The explosion in basic and translational cancer research in recent years has demonstrated that individual cancer genomes are unique and PCR harbour multiple mutations1. With the boom in the development of therapeutics targeted at specific mutations, it is clear that to adequately stratify patients to the appropriate drug or a cocktail of drugs – high quality molecular diagnostics with the ability to provide robust, precise information about multiple genes in a single assay will be crucial. It will be particularly important in the design of rational clinical trials of new therapeutics, so that targeted drugs are tested against tumours which basic research predicts will respond, thus perhaps accelerating the licensing of new cancer treatments. Furthermore, such an assay is going to have to work efficiently on real-life, often heterogeneous archived tissue biopsies and cytological specimens. Assays that can cope with these demands have been used recently in studies from leading North American institutions6,7 and an exciting new initiative in the UK funded by Cancer Research UK has been launched with a similar objective8.
In one of the studies referred to above, Dias-Santagata et al listed four desirable criteria for an assay for human cancer specimens for the delivery of personalised cancer medicine. They suggested it should:
- Be multiplex – multiple loci should be interrogated simultaneously
- Perform well with formalin-fixed paraffinembedded (FFPE) tissue, since virtually all institutions store biopsies in this format
- Be sensitive enough to detect rare mutations
- Have a quick turnaround time (one to two weeks)
In addition to these attributed, it would be ideal if an assay could simultaneously quantify the relative number of copies of mutant to wild type alleles at specific genetic loci; and to be able to accurately quantify somatic copy-number mutations.
Multiplex assays
There are examples of multiplexed techniques that fulfil many of the criteria described above. In their study, Dias-Santagata assessed 120 mutations in 13 genes in a mixed panel of FFPE cancers6; for many of these mutations, specific biological therapeutics exist or are in clinical development. They used the two-stage SnapSHOT protocol consisting of a multiplex PCR strategy, with the second stage being a single base extension sequencing reaction with fluorescently labelled nucleotides. The authors were able to multiplex 10 loci on a standard input DNA of 20ng, although they noted 5ng was sometimes used instead of 20ng if DNA was scarce. An alternative approach is to use the MassARRAY system published by a group from the Dana Farber Cancer Institute7. Mass spectrometry is used to discriminate mutations on amplified PCR products that are put through an allele specific extension reaction. In published work, up to 400 mutations in 33 oncogenes or tumour suppressor genes have been assayed successfully. The recommended input DNA required for this protocol is 250 ng.
These studies are encouraging and represent significant developments in the delivery of personalised medicine. The SnaPshot protocol has the benefit of using technology that is readily accessible, including in diagnostics laboratories internationally. Mass spectrometry is less widely available, although self-contained user-friendly hardware is now available. The only other concern for both methods in some clinical scenarios is the DNA requirement – 250 ng from FFPE is readily achievable in surgical resection specimens, but it may often be more challenging in heterogeneous diagnostic biopsy specimens. In many cases, patients are not scheduled to undergo surgery, at least not in the first instance.
Detection of rare mutations
The sensitivity of a protocol to detect rare mutational events is of great current interest, although there is not yet sufficient data to fully understand their clinical impact. It is not possible to detect subclonal amplification events with PCR but rare sequence mutations can be detected. An unanswered question in oncology is what proportion of cancer cells should harbour a mutation before that mutation is ‘actionable’, that is merits specific treatment. Ultra sensitive techniques on high percentage cancer cell biopsies may well yield multiple actionable mutations, but the clinical benefit of treating a low frequency mutation is not known. This should be a focus of future translational work and may even explain some of the variations in response to targeted treatments currently seen in individuals with identical mutations. I will illustrate the importance of being able to detect rare mutations with three scenarios.
Firstly, many cytological specimens are heterogeneous and cancer cells may account for a very low proportion of the DNA extracted. In this instance, an assay needs to be sensitive and qualitative rather than quantitative, as the percentage of alleles that are mutated is not meaningful if there is significant heterogeneity in the specimen.
A second scenario is when a biopsy comprises of mainly cancer cells (>70 per cent). In the case of lung ADC with a majority clone harbouring an EGFR-TKI sensitive mutation, there may be a rare subclonal population with a mutation – for example EGFR T790M – that confers resistance to the drug. It will be valuable clinically to be able to detect such mutations, if present, at the time of diagnosis.
A third is when the mutation of interest is extremely rare in the sample tested, for example the detection of cancer-related mutations in DNA extracted from peripheral blood.
In each of these cases, it could be argued that the more sensitive the method, the better and that improved sensitivity for rare variants is required methods. The truth is likely to be much more nuanced and it will be important for research into this issue that mutational data is both qualitative and quantitative.
The performance of current protocols in the detection of rare mutations is variable – it has been repeatedly demonstrated that direct sequencing can only detect a mutation if it accounts for a minimum of 20 – 30 percent of the alleles present in a sample. The detection sensitivity of alternative protocols such as pyrosequencing or high-resolution melting curve analysis and the multiplex methods described above is of the order of 5 – 10 per cent. Many groups routinely use allele-specific PCR, for example, with Scorpions probe technology in a real-time PCR protocol9 for detecting mutations in EGFR and KRAS. This approach can detect rare mutations at a frequency of one percent of background normal alleles10.
Specialised PCR protocols have been devised with the aim of enriching for rare variants prior to downstream analysis. This topic, and the many protocols described have recently been reviewed in detail11. I will highlight two protocols – locked nucleic acid (LNA)-PCR makes use of advances in synthetic oligonucleotide chemistry that improve duplex formation and promote mismatch detection. By combining LNA primers with a wild type targeted peptide nucleic acid clamps (PNA) there is preferential amplification of the mutated allele12. A second protocol, COamplification at Lower Denaturation temperature (COLD-PCR) was recently described13. It exploits the observation that heteroduplexes between mutant and wild type sequences will have a slightly lower melting temperature than wild type homo – duplexes. By careful thermal titration of the denaturing phase of the PCR cycle, preferential amplification of the mutant allele is achieved. This has been used successfully on FFPE-derived DNA and with many of the commonly used downstream applications. A particular advantage of the COLD-PCR approach is that unknown as well as known mutations can be detected. It is not yet clear whether this relatively new protocol will be readily adapted to a multiplex assay.
Digital PCR
Digital PCR makes use of the ability of PCR to amplify and thus detect single molecules after DNA has undergone limiting dilution into multiple aliquots. The aliquots of DNA can be held in PCR reaction plates (usually 96-384)14-16 microfluidic chips (hundreds)17, emulsion PCR on beads BEAMing18 or in microdroplets19 (many thousands). There are a number of potential advantages associated with digital PCR.
First, digital assays give precise, easily interpreted relative quantitation of the locus being assessed. This can be exploited to deliver accurate measurement of the percentage of mutated alleles at a locus and to interrogate somatic copy-number variants18,20. This type of integrated analysis may prove to be of considerable importance in personalised cancer medicine. A second advantage is that the sensitivity and ability to detect rare variants is high and increases with the number of aliquots. Digital PCR has already been shown to offer ultra-high sensitivity for rare variants, with a detection sensitivity of 0.01 per cent mutant alleles reported18 so it may be an ideal approach for the detection of rare mutations in noninvasive specimens such as peripheral blood. Third, as limiting dilution PCR is being performed, the template requirements can be very low – this may be of critical importance in the drive to deliver multilocus personalised medicine on diagnostic biopsies21. Our own current emphasis is on optimising the μMCC protocol16 so that an integrated analysis of hundreds of loci for both CNVs and sequence mutations will be possible on small heterogeneous FFPE biopsies.
A number of platforms for digital PCR are available17 or are in development19,22. The number of aliquots influences the precision of quantitation, the dynamic range over which quantitation is accurate and the sensitivity to detect rare mutations.
Next Generation Sequencing
This article has concentrated on PCR as a tool to deliver personalised medicine. However, a major revolution in the analysis of the cancer genome over the last few years has been the application of next generation sequencing (NGS) protocols that can provide an unparalleled insight into individual cancer genomes. It may well be that the performance and analysis costs of NGS or some of the available single molecule sequencing protocols will fall sufficiently for them to be considered a routine part of the cancer work-up in the future.
For now, it is important to stress the synergies between PCR and NGS technologies. It is, of course, the case that PCR is central to NGS protocols, but it is also increasingly clear that in terms of personalised cancer medicine, there are exciting possibilities for PCR and NGS protocols to complement each other. For example, PCR is a target enrichment strategy for downstream sequence analysis on NGS platforms23-25. Multiple target sequences in cancer-related genes can be amplified and the capacity of NGS is such that multiple genes from multiple patients could easily be simultaneously analysed in a single run. The primers used can be designed with barcodes so that amplicons from individual patients can be readily identified. A recent paper described how PCR and NGS techniques may be complementary in the non-invasive monitoring of cancer. Using NGS translocations specific to individual tumours were identified26 and it was possible to design primer sets specific to probe for these rearrangements. These tumour-specific primer sets were then used in ultrasensitive digital PCR assays to quantitate the abnormal translocation in peripheral blood plasma.
Although this is a fast moving field, it seems a reasonable assumption that the polymerase chain reaction will, for some time to come, remain central to efforts to intelligently guide cancer therapeutics based on the molecular events detected in individual cancers.
References
- Stratton MR, Campbell PJ, Futreal PA. The cancer genome. Nature 2009;458:719-724; DOI nature07943 [pii] 10.1038/nature07943.
- Sholl LM, Yeap BY, Iafrate AJ, Holmes-Tisch AJ, Chou YP, Wu MT et al. Lung adenocarcinoma with EGFR amplification has distinct clinicopathologic and molecular features in never-smokers. Cancer Res 2009;69:8341- 8348; DOI 0008-5472.CAN-09-2477 [pii] 10.1158/0008-5472.CAN-09-2477.
- Campbell PJ, Pleasance ED, Stephens PJ, Dicks E, Rance R, Goodhead I et al. Subclonal phylogenetic structures in cancer revealed by ultra-deep sequencing. Proc Natl Acad Sci U S A 2008;105:13081-13086; DOI 0801523105 [pii] 10.1073/pnas.0801523105.
- Eberhard DA, Johnson BE, Amler LC, Goddard AD, Heldens SL, Herbst RS et al. Mutations in the epidermal growth factor receptor and in KRAS are predictive and prognostic indicators in patients with non-small-cell lung cancer treated with chemotherapy alone and in combination with erlotinib. J Clin Oncol 2005;23:5900-5909.
- NICE. National Institute for Health and Clinical excellence 2010. http://www.nice.org.uk.
- Dias-Santagata D, Akhavanfard S, David SS, Vernovsky K, Kuhlmann G, Boisvert SL et al. Rapid targeted mutational analysis of human tumours: a clinical platform to guide personalized cancer medicine. EMBO Mol Med 2010;2:146-158; DOI 10.1002/ emmm.201000070.
- MacConaill LE, Campbell CD, Kehoe SM, Bass AJ, Hatton C, Niu L et al. Profiling critical cancer gene mutations in clinical tumor samples. PLoS One 2009; 4:e7887; DOI 10.1371/ journal.pone.0007887.
- Callaway E. Cancer-gene testing ramps up. Nature 2010;467:766-767.
- Whitcombe D, Theaker J, Guy SP, Brown T, Little S. Detection of PCR products using self-probing amplicons and fluorescence. Nat Biotechnol 1999;17:804-807; DOI 10.1038/11751.
- Board RE, Thelwell NJ, Ravetto PF, Little S, Ranson M, Dive C et al. Multiplexed assays for detection of mutations in PIK3CA. Clin Chem 2008;54:757-760; DOI clinchem.2007.098376 [pii] 10.1373/clinchem.2007.098376.
- 11. Milbury CA, Li J, Makrigiorgos GM. PCR-based methods for the enrichment of minority alleles and mutations. Clin Chem 2009;55:632-640; DOI clinchem.2008.113035 [pii] 10.1373/ clinchem.2008.113035.
- Nagai Y, Miyazawa H, Huqun, Tanaka T, Udagawa K, Kato M et al. Genetic heterogeneity of the epidermal growth factor receptor in non-small cell lung cancer cell lines revealed by a rapid and sensitive detection system, the peptide nucleic acid-locked nucleic acid PCR clamp. Cancer Res 2005;65:7276-7282; DOI 65/16/7276 [pii] 10.1158/0008-5472.CAN-05-0331.
- Li J, Wang L, Mamon H, Kulke MH, Berbeco R, Makrigiorgos GM. Replacing PCR with COLD-PCR enriches variant DNA sequences and redefines the sensitivity of genetic testing. Nat Med 2008;14:579-584; DOI nm1708 [pii] 10.1038/nm1708.
- Vogelstein B, Kinzler KW. Digital PCR. Proc Natl Acad Sci U S A 1999;96:9236-9241.
- Daser A, Thangavelu M, Pannell R, Forster A, Sparrow L, Chung G et al. Interro gation of genomes by molecular copy-number counting (MCC). Nat Methods 2006;3:447-453.
- McCaughan F, Darai-Ramqvist E, Bankier AT, Konfortov BA, Foster N, George PJ et al. Microdissection molecular copy-number counting (microMCC)–unlocking cancer archives with digital PCR. J Pathol 2008;216:307-316.
- Fluidigm. http://www.fluidigm.com 2010.
- Li M, Diehl F, Dressman D, Vogelstein B, Kinzler KW. BEAMing up for detection and quantification of rare sequence variants. Nat Methods 2006;3:95-97; DOI nmeth850 [pii] 10.1038/nmeth850.
- QuantaLife. http://www.quantalife.com/2010.
- Wang J, Ramakrishnan R, Tang Z, Fan W, Kluge A, Dowlati A et al. Quantifying EGFR alterations in the lung cancer genome with nanofluidic digital PCR arrays. Clin Chem 2010;56:623-632; DOI clinchem.2009.134973 [pii] 10.1373/clinchem.2009.134973.
- McCaughan F, Dear PH. Single-molecule genomics. J Pathol 2010;220:297-306; DOI 10.1002/path.2647.
- Inostics. http://www.inostics.com/2010.
- Mamanova L, Coffey AJ, Scott CE, Kozarewa I, Turner EH, Kumar A et al. Target-enrichment strategies for next-generation sequencing. Nat Methods 2010;7:111-118; DOI nmeth.1419 [pii] 0.1038/nmeth.1419.
- Johansson H, Isaksson M, Falk Sorqvist E, Roos F, Stenberg J, Sjoblom T et al. Targeted resequencing of candidate genes using selector probes. Nucleic Acids Res 2010; DOI gkq1005 [pii] 10.1093/nar/gkq1005.
- Meyer M, Kircher M. Illumina sequencing library preparation for highly multiplexed target capture and sequencing. Cold Spring Harb Protoc 2010;2010:pdb prot5448; DOI 2010/6/pdb.prot5448 [pii] 0.1101/ pdb.prot5448.
- Leary RJ, Kinde I, Diehl F, Schmidt K, Clouser C, Duncan C et al. Development of personalized tumor biomarkers using massively parallel sequencing. Sci Transl Med 2010;2:20ra14; DOI 2/20/20ra14 [pii] 10.1126/scitranslmed.3000702.
About the Author
Frank McCaughan graduated in medicine from the University of Edinburgh and completed his postgraduate training as a respiratory physician in London. During this training period, he became closely involved with the Early Lung Cancer Project at University College London Hospital. He obtained his PhD from University College London while working on the early genomic events that are key to the development of squamous lung cancer. He has several years experience in single molecule digital PCR strategies and currently is focused on two research themes, the pathogenesis of squamous lung cancer and developing digital PCR strategies for patient stratification in lung adenocarcinoma.
Issue
Related topics
DNA, Next Generation Sequencing, Personalised medicine, Polymerase Chain Reaction (PCR)