Continuous flow processing in the pharma industry – an unstoppable trend?
Posted: 22 October 2015 |
Continuous flow processes have many distinct advantages over discontinuous batch production and therefore, in the last century, continuous operation has become by far the most dominant form of production for high-volume and low-cost materials such as petrochemical and commodity chemicals. The first applications of continuous processes in the pharmaceutical industry emerged only comparatively recently and the vast majority of production is still undertaken in batch reactors. Herein, we highlight some of the advantages that continuous flow processing offers for the synthesis of pharmaceuticals and fine chemicals.
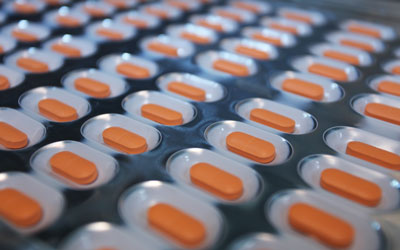
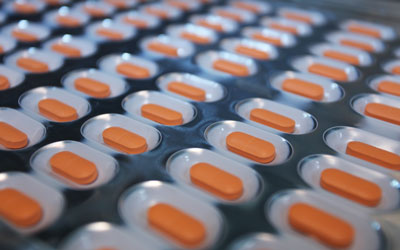
Drivers for the pharmaceutical industry to move production from batch to flow are much weaker than for the commodity chemical industry. The production volumes of pharmaceuticals are considerably less than those of bulk chemicals and product lifecycles are often short. Furthermore, pharmaceuticals are significantly more complex than commodity chemicals and their production usually requires many – often widely diverse – synthesis steps in addition to multiple rounds of isolation and purification. The complexity and diversity of these molecules, and the consequently involved and diverse process conditions required for their synthesis and isolation demand flexible, multipurpose reaction vessels for their production. Stirred tank-reactors are easy to handle and can be employed for a range of different operations. However, despite their long history and prevalence in synthesis laboratories, batch-type reactors have some severe, well-recognised limitations. Most importantly, chemical reactions which release large amounts of energy or proceed via unstable, highly toxic or explosive intermediates are difficult or impossible to implement in tank reactors.
In the past few decades, technologies for continuous synthesis have advanced tremendously and a plethora of discrete flow components and modules, including pumps, mixers, reactors and separation units, along with an increasing number of integrated standalone flow reactors, have become commercially available. The emergence of various reactor designs, addressing the diverse physicochemical requirements of chemical reactions, along with the emergence of technologies for feed delivery, flow metering, continuous separation, etc., enables the assembly of specialised, high-performance flow systems by combining these operation units.
The modular approach provides the flexibility required for fine chemical synthesis while the advantages inherent to continuous production are maintained. It is partly because of the commercial accessibility of flow equipment for organic synthesis on small to medium scales that flow chemistry evolved from a pure research topic in chemical engineering to an almost ubiquitously applicable technique in organic synthesis laboratories.Furthermore, the pharmaceutical industry is investing heavily in the development and advancement of continuous manufacturing. In fact, virtually all large pharmaceutical companies now have dedicated groups working on the development and implementation of flow synthesis. Indeed, a 2005 roundtable consisting of 15 pharmaceutical companies and contract manufacturers identified continuous processing as one of the “key green engineering research areas for sustainable manufacturing”.1
Miniaturisation provides enhanced heat/mass transfer and safety
It was estimated that around a half of the reactions currently run in the pharmaceutical industry could benefit from being performed in flow. Perhaps the foremost advantage of flow synthesis is the enhanced safety profile.2 Since product is formed continuously, with no downtime of the production for charging and discharging the reactor, large amounts of material can be processed even in comparatively small reactors. In fact, the actual reactor units typically have channel diameters ranging from below 100µm (i.e., microreactors) to few millimeters (meso/milli-reactors). Whereas tank reactors with volumes of tens of cubic meters are common in chemical companies, the internal volumes of flow reactors typically range from below 1µL to a couple of liters. A smaller volume implies that there is less material in the reactor and the amount of material or energy released in a safety event is correspondingly reduced. In fact, the material content in microreactors is often too small to cause serious damage to human health or the environment in the event of an accident. Thus, continuous manufacturing enables reactions to be performed or reaction conditions to be applied which would be infeasible or nearly impossible in batch mode on larger scales.2
Short atom- and step- efficient synthetic routes frequently involve the use of highly reactive or otherwise hazardous compounds, and, in the last decade, a large amount of work was directed towards adapting these reactions for continuous processing. Continuous flow variants have been developed for a plethora of notoriously hazardous transformations, including nitration reactions, reactions with azides, phosgene, cyanides, isocyanides, diazo- and diazonium compounds.2 For instance, a chemist from DSM developed a pilot scale continuous flow nitration plant for the generation of the nitrate-building block in the non-steroidal anti-inflammatory drug Naproxcinod (nitronaproxen , NicOx) (Scheme 1a; page 00).3 The reaction was performed with neat nitric acid in Corning glass reactors with an internal volume of below 150mL. One reactor module delivered about 13kg/h of the product. Parallelisation of the modules afforded a production unit with a capacity to produce 100kg/h of product, corresponding to an annual productivity of 800t.3
Crucial for reactions involving very reactive reagents or intermediates is the exceptionally fast heat and mass transfer which can be accomplished in microstructured devices.2 For fast or highly exothermic reactions, mass and heat transfer become limiting and the reaction mixture has to be cooled or diluted to reduce the reaction rate and to keep the reaction as uniform as possible, or reagents have to be dosed slowly to the mixture to maintain temperature at a constant level. Adequate mixing and dissipation of excess energy becomes increasingly difficult upon scale-up in stirred tank reactors and accumulation of heat or formation of hot-spots might diminish reaction selectivity and can lead to serious risks of thermal runaways. In microstructured reactors, mixing can be achieved on time scales of milliseconds. Similarly, considerably improved heat exchange efficiency is obtained due to the small channel dimensions and the very large surface-to-volume ratios of microreactors. Thus, fast reactions, such as reactions involving organometallic compounds like alkyl lithium or Grignard reagents, can be run at significantly higher temperatures than usual, often near, or even at, room temperature.2 The use of cryogenic temperatures is rendered unnecessary and these reactions become economically and substantially more attractive.
Process intensification
On the other hand, high temperatures might be applied deliberately to increase the reaction rate.2 The reaction rate usually depends roughly exponentially on temperature and increasing the reaction temperature is often the easiest and most effective way to enhance reactivity. However, temperatures above around 200°C are largely outside the operation range of most conventional tank reactors in the pharmaceutical industry. Furthermore, for batch reactions the whole installation has to be heated to the target temperature and cooled after the reaction is finished. In contrast, for flow processes only the reaction mixture is heated while it is carried through the channels of a flow reactor at constant temperature, and the processed mixture is subsequently cooled in a heat exchanger at constant temperature.
Combined with the rapid heat exchange capability of microreactors, extremely efficient and responsive heating is thus attained. Furthermore, the small dimensions of microreactors entail high resistance to pressure. As a result, temperatures far above the atmospheric boiling point of the solvent become accessible fairly easily and safely. Higher reaction rates, in turn, yield higher throughputs or allow a further reduction of the reactor size and consequently reactor cost. Since the advent of microwave reactors around 50 years ago it became obvious that a wide range of diverse chemical transformations are very amenable to high temperature processing. Continuous flow microreactors now provide the technology necessary to scale these reactions up to industrially relevant scales.2
Reactions with gaseous reagents
Continuous flow microreactors present an ideal platform for reactions with gaseous reagents. Gases can be dosed into the flow system with precise stoichiometry using mass flow controllers and intense mixing of the gaseous and liquid phase can be achieved. Furthermore, high pressure operation increases the concentration of the gas in the liquid phase. Combustion and explosion hazards are reduced in channels of small diameter and, consequently, reactions can be performed under unusually harsh process conditions in a safe and controllable manner. In particular, continuous flow hydrogenations have found extensive application in the chemical industry, and, with the introduction of commercial bench-top high-pressure hydrogenators, it has been rapidly spread in synthesis laboratories.2
The synthesis of the penultimate intermediate of Eli Lilly’s product, LY500307, a potent, selective oestrogen receptor β agonist, required an asymmetric rhodium catalysed hydrogenation in the presence of a Josiphos ligand (Scheme 1b; page 00).4 Pressures up to 70 bar were necessary to reduce substrate-to-catalyst loading to economically viable levels. For the pilot-scale reaction, a 73L stainless steel tube reactor was employed. The asymmetric hydrogenation was coupled with a continuous downstream work-up to provide the desired product with more than 99% ee. In addition to safety benefits, the capital cost for the flow plant was significantly less and it was considerably faster to build than a high pressure hydrogenation autoclave.4
Continuous multistep synthesis
For a flow process, multiple reactor and separation modules can be arranged consecutively to form a single continuous operation unit. For continuous multistep synthesis, reagents are fed into the assembly at specified points and the reaction stream is manipulated along the reaction path to suit the needs of individual transformations. Reaction intermediates are thus generated and directly consumed in the closed and, where necessary, pressurised reaction environment of the microreactor. In recent years, considerable efforts have been undertaken to devise uninterrupted multistep flow reaction sequences, and elaborate processes for the direct synthesis of complex molecules from simple starting materials have been demonstrated. However, though impressive, the benefits of these complex processes and the practicability for medium to large synthesis have not yet been established. Design and development of multistep continuous processes is certainly challenging and requires a detailed understanding of the individual operations and a careful, ‘holistic’ planning of the whole reaction sequence. An obvious advantage arises when a multistep sequence eliminates the need to isolate explosive or toxic intermediates.2
None-routine chemistry
Continuous flow and microreactor technology provides new opportunities for none-routine chemistry such as electro- and photochemistry. These methods are traditionally hardly considered when a route to a new molecule is designed, even though transformations are enabled which are difficult to achieve by mainstream chemistry. In recent years research in continuous flow photochemistry in particular has become very active. Many challenges of photochemistry, including the exponential decrease of the photochemical efficiency with the path length (Beer-Lambert law), are naturally addressed by microreactors. A striking example was demonstrated by chemists from the Max-Planck Institute for Colloids and Interfaces, Germany (Scheme 1c; page 00).5 The group demonstrated a fully continuous synthesis of artemisinin, currently the most effective antimalarial drug. The key step in the synthesis of artemisinin is an ene reaction of dihydroartemisinic acid with singlet-oxygen. The photo-generation of singlet oxygen in a continuous flow photoreactor and the concomitant ene reaction was directly coupled with the cleavage of the oxygen-oxygen bond as well as subsequent oxidation with triplet oxygen and condensation to artemisinin in a tube reactor. Pure artemisinin was obtained in 46% overall yield after a total residence time of only 12 minutes in the continuous flow system.5
Downstream processing
The actual chemical transformation in the synthesis of a pharmaceutical frequently consumes only a fraction of the materials, time and energy expended. About two to three separation operations are commonly performed for every reaction step. The vast majority of these separation steps are currently performed in batch or semi-batch equipment. However, for flow processes to deliver the projected benefits to the pharmaceutical industry, the whole generation of the final, purified pharmaceutical product from simple synthetic precursors ultimately needs to be continuous. Many work-up processes, such as distillation, extraction or phase separations, are reasonably easily adapted for continuous processing and are often considerably more effective when run continuously. Novel, continuous, and intensified techniques are presently developed for challenging separation operations including filtration, washing and drying. The Novartis–MIT Center for Continuous Manufacturing recently disclosed the continuous end-to-end production of Tekturna (aliskiren, Novartis) a drug used to treat high blood pressure (Scheme 2; page 00).6 The fully continuous process included all synthetic steps, separations, crystallisations, drying and formulation to produce the final tablets. The number of unit operations was reduced from 21 to 14, and the total residence time was an order of magnitude shorter than the production time needed for the batch process.6
Conclusion
In 2011 the US Food and Drug Administration predicted that in the next 25 years “continuous manufacturing will make current methods obsolete”.7 However, even though continuous processing offers many rather clear advantages over traditional batch-wise operation, this technique has yet to become routinely used in the pharmaceutical industry. The vast majority of operations in the pharmaceutical industry are still run in stirred tank reactors. The development of a continuous flow synthesis is engineering intensive and, additionally, requires a detailed understanding of the involved chemical processes. Furthermore, although many of the presently performed batch operations could be translated to continuous processes fairly easily, without major modifications or re-optimisation, there is little incentive to do so in the presence of sufficient capacity in the existing batch installations.
Perhaps the true potential of continuous manufacturing will be realised when a reaction sequence is planned from the very outset with the unique opportunities enabled by this technology in mind. Chemistry which was impractical or simply impossible to run in batch reactors can be contemplated and entirely new synthesis routes become accessible. To fully establish continuous flow processes in the pharmaceutical industry, further development and maturation of the technology, as well as a continuous commitment of chemists and engineers to design ever more efficient and sustainable processes, will be required.8
References
- (a) Poechlauer, P, Colberg, J, Fisher, E, et al. Pharmaceutical roundtable study demonstrates the value of continuous manufacturing in the design of greener processes, Org. Process Res. Dev. 2013; 17, 1472-1478; (b) Poechlauer, P, Manley, J, Broxterman, R, et al. Continuous processing in the manufacture of active pharmaceutical ingredients and finished dosage forms: an industry perspective, Org. Process Res. Dev. 2012; 16, 1586-1590; (c) Jiménez-González, C, Poechlauer, P, Broxterman, QB, et al. Key green engineering research areas for sustainable manufacturing: a perspective from pharmaceutical and fine chemicals manufacturers, Org. Process Res. Dev. 2011; 15, 900-911
- Gutmann, B.; Cantillo, D.; Kappe, C. O. Continuous-Flow Technology—A Tool for the Safe Manufacturing of Active Pharmaceutical Ingredients, Angew. Int. Ed. 2015; 54, 6688–6728
- Braune, S, Poechlauer, P, Reintjens, R, et al. Selective nitration in a microreactor for pharmaceutical production under cGMP conditions, Chim. Oggi/Chem. Today. 2009; 27(1), 26-29
- Johnson, M. D.; May, S. A.; Calvin, J. R.; et al. Development and scale-up of a continuous, high-pressure, asymmetric hydrogenation reaction, workup, and isolation, Org. Process Res.Dev. 2012; 16, 1017–1038
- Kopetzki, D, Lévesque, F, Seeberger, PH. 2013; A continuous-flow process for the synthesis of artemisinin, Chem. Eur. J., 19, 5450–5456
- Heider, PL, Born, SC, Basak, S, et al. Development of a Multi-Step Synthesis and Workup Sequence for an Integrated, Continuous Manufacturing Process of a Pharmaceutical, Org. Process Res. Dev. 2014; 18, 402-409
7) http://www.in-pharmatechnologist.com/Processing/Continuous-manufacturing-will-make-current-methods-obsolete-FDA-says
8) Baxendale, IR, Braatz, RD, Hodnett, B, et al. Achieving Continuous Manufacturing: Technologies and Approaches for Synthesis, Workup, and Isolation of Drug Substance May 20–21, 2014 Continuous Manufacturing Symposium. 2015; J. Pharm. Sci. 104, 781-791
Biographies
Bernhard Gutmann studied Chemistry at the University of Graz, Austria. In 2009 he obtained his diploma degree in the field of microwave assisted organic synthesis. He continued with PhD work in the group of Professor C. Oliver Kappe on projects related to continuous flow processing. After receiving his PhD degree in 2013, he joined the Christian Doppler Laboratory for Continuous Flow Chemistry at the University of Graz and is currently Senior Researcher at the Research Center Pharmaceutical Engineering (RCPE) where his research is focused on the continuous-flow synthesis of APIs.
Christian Oliver Kappe is Professor of Chemistry at the University of Graz and Key Researcher at the Research Center Pharmaceutical Engineering (RCPE) in Graz, Austria. He received his undergraduate and graduate education at the University of Graz. After periods of postdoctoral research work at the University of Queensland and at Emory University, he moved back to the University of Graz in 1996 to start his independent academic career. He became Associate Professor in 1999 and was appointed Full Professor in 2011. The co-author of ca. 350 publications, his main current research interests are related to continuous flow chemistry, API manufacturing and process intensification. He is Editor-in-Chief of the Journal of Flow Chemistry, short-course instructor on continuous flow technology and a consultant for the pharmaceutical industry.