Imaging for decision-making in drug discovery and early development
Posted: 23 December 2014 |
The cost of drug discovery and development, depending upon the size of a given company, has been estimated upwards of $5 billion. Hay et al. recently published a review of clinical development success rates showing only a 10.4% likelihood of regulatory approval of all drugs entering Phase I, 64.5% of which make it to Phase II where the likelihood of regulatory approval increases to only 16.2%.
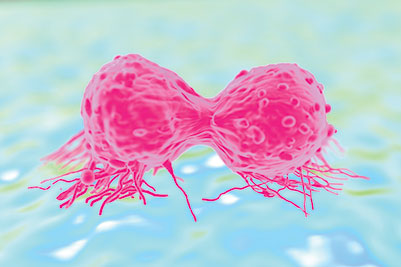
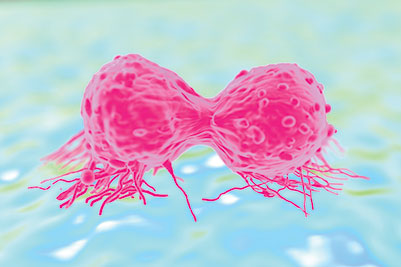
However, using a “fail fast, fail cheap” approach can help address the cost challenges and alarming failure rates. This means improving the decision-making process for compounds approaching Phase I trials, making better use of Phase I studies for decision-making beyond safety, and improving the quality of compounds entering the clinic.
In this review, we highlight a few of the ways in which Eisai is attempting to address the research and development bottleneck by adding fit-for-purpose imaging biomarkers to discovery and early development programs for decision making. Use of biomarkers, including imaging, has increased over the past decade with the hopes of improving the quality of compounds and decision-making in early development. Meanwhile, a recent publication by Cook et al.3, which presents a postmortem of AstraZeneca programs from 2005-2010, summarises what they found to be the “five most important technical determinants of project success and pipeline quality”, namely “the right target, the right patient, the right tissue, the right safety and the right commercial potential,”. Imaging biomarkers can significantly contribute to these determinants, making imaging a necessary tool in the decision-making process as opposed to “nice to have” biomarkers.
Imaging can provide critical and impactful data necessary to drive decision-making in modern drug discovery programs. It can also provide unique information beyond traditional methodology and leverage the strengths of non-invasive, in vivo assessment of biological processes. Throughout the development pipeline, imaging may be used to inform on molecular target engagement, proof-of-mechanism, biodistribution, pharmacokinetics, pharmacodynamics, efficacy, stratification and even off-target activity or drug safety. Successful implementation of imaging requires a consideration of a number of factors including choice of imaging modality/biomarker, chemistry, preclinical animal models, novel versus established methodology, and integration with traditional biomarkers to provide actionable data to project teams. Below we provide case studies supporting preclinical and translational decision making.
The right patient: using radiolabeled antibodies
Antibody, peptide, and antibody-drug conjugates (ADC) therapies are increasingly being investigated owing to their ability to better target specific mutations or targets within diseased tissue, typically cancer, where they can have a direct effect on the tumour, indirectly affect the tumour by recruiting the immune system, or deliver radio- or chemotherapies. Both preclinically and clinically, it is essential to demonstrate target engagement. Imaging of a radiolabeled antibody allows for patient selection, stratification throughout development and potential use as a companion diagnostic4 such as that being explored in folate targeted therapies5. Figure 2 shows SPECT images in a xenograft mouse model five days after administration of a 131-indium radiolabeled antibody targeted to the tumour, compared to a non-specific antibody.
The right target: evaluating efficacy through imaging
In considering the ideal target, imaging efficacy can support preclinical and even clinical decision-making. Examples of this are: osteoporosis imaging of bone mineral density by energy X-ray absorptiometry and computerised tomography (CT) measures of tumour size in drug development or patient treatment of cancer. Assuming there is evidence that a potential therapy has sufficient target engagement, a lack of demonstratable efficacy suggests the target may not be viable. This is especially critical when exploring novel mechanisms of action (MOA), or when MOA is understood but additional therapeutic benefit is identified.
In Figure 3 we show an example of efficacy for a noncompetitive AMPA receptor antagonist (GYKI 52466) before and after dosing in cynomolgus monkeys using 123I-B-CIT SPECT imaging, which maps the distribution of dopamine transporter (as seen on the left) and 123I-IBZM, which maps the distribution of dopamine receptors (as seen on the right). SPECT imaging was able to measure a significant effect of an AMPA receptor antagonist on dopamine receptors. Increased dopamine transport (left) causes competition for available receptors and reduces IBZM signal (right).
The right safety: using imaging to assess toxicity
An interesting area of focus has been the application of imaging towards assessment of drug safety in preclinical programs. The capability of imaging to study the same animal over time, using the animal as its own control, decrease read-out time, examine reversibility, look at in vivo function as well as morphology, and potential of translation can be used with traditional methods to support decision making. Several publications highlight this potential in areas such as foetal bone6, drug induced hepatic steatosis7, prostate histopathology8 and vascular microhemorrhages9. Eisai has an exploratory program for the use of drug safety imaging biomarkers for both specific use applications as well as general screening of advanced discovery programs.
The right tissue, safety and patient in early clinical development
The development and application of a positron emission tomography (PET) tracer is one the more powerful applications of imaging in support of neuroscience clinical programs, having the ability to save time, reduce cost, and speed up the development of new chemical entities while allowing for no go decisions. Provided adequate preclinical and Phase 0 tracer characterisation, an application can be made to consider a Phase I study to evaluate target engagement of the novel compound using the previously characterised PET ligand. A receptor occupancy study establishes the relationship between receptor occupancy and plasma concentration of a compound. With this relationship, it becomes fairly easy to use established pharmacokinetic information of a compound to assess the degree of target engagement that will be expected. In cases where chronic dosing with a long plasma half-life compound leads to accumulation, a lower dose of compound could be used to maintain the target concentration and mitigate potential side effects. Studies of PET tracers in this capacity have been carried out previously, allowing investigators to optimise dosing and to increase the therapeutic window10, 11,12. Furthermore, obtaining a plasma concentration/target occupancy relationship has led to informed development termination decisions resulting from side effects in the dosing window12,13. In addition, PET receptor occupancy studies have been able to demonstrate the situation when a target supported by preclinical studies is not available or present when studied in a patient population. An example is the evaluation of the neurokinin 1 receptor antagonist (NK1) for depression. The NK1 antagonist, aprepitant, was shown to have no beneficial effect in patients with depression. A receptor occupancy study demonstrated that the dose used resulted in a greater-than 95% receptor occupancy, allowing one to argue against a lack of plasma exposure as the rationale for obtaining a negative outcome. This finding gave the company the information to obtain a no go decision for the compound, thus saving time and costs14. Fortunately, a similar study was able to demonstrate efficacy in chemotherapy-induced nausea and vomiting, and thus enable the registration of Emend10,15. In the area of neuroscience, PET imaging is an important decision driver in drug discovery and development.
The right safety and patient in early clinical development
Another significant opportunity to apply imaging for decision making in early development is the use of [18F] FDG (fluorodeoxyglucose)-PET for oncology programs. Most cancer cells metabolise glucose at a significantly higher rate than normal cells16. As an analog of glucose-accelerated uptake and hexokinase-mediated metabolism, FDG can be used to noninvasively measure metabolism in cancer cells. Because FDG uptake reflects the underlying metabolism of cancer cells, this effect is independent of the mechanism of action of the therapeutic compound. This has allowed for the investigation of compounds that target diverse mechanisms of action such as cKIT inhibitors, EGFR inhibitors, angiogenesis inhibitors, tyrosine kinase inhibitors, and several others. FDG is an important imaging biomarker for new therapeutic approaches, especially where other accessible biomarkers may not be available.
Wahl et al.17 first observed that a reduction in FDG signal can be used as an early indicator of later clinical response to chemotherapy treatment and over the last decade the FDG early response has been explored extensively in both clinical care and clinical trials. Since 1990, the use of FDG-PET in detection, reporting of evaluation and assessing an early indication of response to anti-tumour agents has increased annually (Figure 4). A decade ago, W. Weber18 reviewed the use of FDG-PET in assessing the response of tumours to chemotherapy and concluded that there was considerable evidence in the literature that FDG-PET can be used to assess tumour response to chemotherapy and chemoradiotherapy. However, the uptake and adoption of this method in clinical trials has been relatively conservative despite its potential to speed-up the delivery of new anticancer agents to patients.
In the current age of targeted therapies where tumour stasis has been demonstrated to be beneficial to patients along with tumour size reduction, anatomical measures of tumour size are less predictive of clinical outcome. Cytostatic agents are not as amenable to the established methods of assessing a surrogate end point of tumour size reduction by CT or MRI since progression-free survival and overall survival can be observed in the absence of a greater-than 20% reduction in the single longest dimension measured; the established criteria for Partial Response by RECIST 1.1. It is with these targeted therapies that the benefits of FDG-PET imaging can be uniquely demonstrated in the clinic. Over the last several years, investigators have been able to identify patients having a metabolic response much earlier in their treatment than was previously available using CT19,20,21. Conversely, and critical to drug discovery and care management, patients that do not respond to a given compound can be identified much earlier in the process21. This allows for removal of patients from studies where they will have no benefit from the assigned treatment. For decision-making, if patients do not show a response to FDG-PET but rather show an increase in FDG-PET signal, this may provide justification of an early no go decision for the compound, likely saving tens to hundreds of millions of dollars in development costs.
Clinical trial costs can also be reduced by using FDG as an early-response indicator by decreasing the number of patients in a trial. Jacene et al22 reported that within an institution, the accuracy and reproducibility of monitoring a change with treatment by FDG-PET was significantly higher than monitoring the response with CT and RECIST. This can translate into shorter clinical trials by powering studies with fewer patients. Consequently, treatments that have a higher likelihood of clinical success can be moved more quickly into patients’ reach.
Also of relevance with targeted therapies is the selection of doses that are studied in Phase II and Phase III clinical trials. Traditionally, the maximum tolerated dose (MTD) of a chemotherapy has been supported by data obtained with chemotoxic agents showing a dose-proportional benefit23 but the risk-benefit ratio has supported doses with an acceptable level of toxicity. With cytostatic agents, which can have a large tolerability range, it may be challenging to determine MTD or may even introduce toxicity by going over an optimal biologic dose (OBD). Two recent statements from members of the FDA have disclosed the issues of using MTD to conduct Phase II and Phase III clinical trials. At Friends of Cancer Research in 2013, R. Pazdur (Director, USFDA Office of Hematology and Oncology Products) said “We have accepted too high a degree of toxicity in cancer drugs and it has become a problem. Drug companies don’t want to do Phase II dosing studies to determine whether the maximal tolerated dose is the optimal dose. We simply don’t have the license to cause patients so much toxicity”24. In his presentation, Pazdur described data showing a lower dosing regimen gave similar results as an MTD. This idea was reiterated by T. Prowell (Breast Cancer Scientific Lead at the FDA) who in describing some of the issues plaguing oncology drug development said “drug developers have a tendency to move forward with the maximally tolerated dose even though it is not clear it is necessary or appropriate for targeted drugs”25. In support of this, Jain et al26 reviewed 24 oncology trials at MD Anderson Cancer Center and found a reduction in dose down to levels of 25% of the MTD provided similar response rates, overall survival and progression free survival but with far fewer side effects26. These combined data suggest it may be necessary in the near future to better justify clinical trial doses and define the optimal dose moving into Phase II.
FDG PET may be used in deciding the optimal biological dose of a targeted therapy. Since FDG provides an early response to treatment, obtaining an optimal dose could be realised by following the response of patients to different doses of the compound under investigation with FDG. In a dose-escalation study, once patients exhibit a change in FDG indicative of early response, this may then be recommended as the optimal biologic dose. Alternatively, after determining MTD, FDG response can be monitored in a dose de-escalation expansion study, reducing dose until the FDG response drops below maximal response. FDG-PET could also be used to identify responsive tumour types for enrichment of later phase trials.
The strategy of using FDG-PET as an early indication of a positive response is not unique within the pharmaceutical industry. An examination of clinical trials from ClinTrials.gov showed an increased number of companies using FDG-PET as endpoints for compounds in Phase I and Phase II (Figure 5). One of the critical factors that is necessary to support this direction is to ensure studies conform to standards that have been set by the European Union and the United States, which provide guidelines for conducting FDG studies27,28. An example of one of these standards is the timing between injection of FDG and scan acquisition at baseline and follow-up scans. Deviation of greater-than 10 minutes in this interval between successive scans can have the effect of changing a partial metabolic response to stable metabolic disease or progressive metabolic disease in certain patients27. Thus FDG-PET provides an early response to targeted oncology therapy, guiding decision-making in drug development. If studies are performed using established guidelines across centers, the potential to find new therapeutic agents to combat cancer could be realised at a quicker pace and with better patient outcomes than has previously been possible.
Discussion
Given the modern challenges facing the pharmaceutical industry, such as the high costs involved, lengthy timelines and low success rates, it is becoming increasingly important to consider opportunities to design and execute studies that directly contribute decision-making data. Imaging, with its potential to reduce costs and inform early decisions, has demonstrated itself invaluable in this process when applied to problems less suited to other methodologies. The examples discussed in this review are not comprehensive, but touch on some of the areas we consider especially suited to imaging biomarkers. Imaging supports decision-making on the basis of target engagement, proof-of-concept, drug safety, patient selection, and dose selection. Together with other biomarkers, imaging is well positioned to accelerate and inform decision-making in the drug discovery and development process and shows real promise in getting the right drugs to patients in need.
References
- Matthew Herper, “The cost of creating a new drug now $5 billion, pushing big pharma to change,” Forbes, August 3, 2013
- Hay et al. Nature Biotech 32:40, 2014
- Cook et al. (Nat Rev Drug Discov. 2014 Jun;13(6):419-31
- Wu AM, Olafsen T: Antibodies for molecular imaging of cancer. Cancer J 14:191-197, 2008
- Leamon C.P. Folate-targeted drug strategies for the treatment of cancer. Curr Opin Investig Drugs. 2008; 9(12):1277-1286
- Winkelmann C.T., Wise L.D. High-throughput micro-computed tomography imaging as a method to evaluate rat and rabbit fetal skeletal abnormalities for developmental toxicity studies (J Pharmacol Toxicol Methods. 2009; 59:156–165)
- Hockings P.D. et al. (2003) Rapid reversal of hepatic steatosis, and reduction of muscle triglyceride, by rosiglitazone: MRI/S studies in Zucker fatty rats. Diabetes, Obesity and Metabolism 5, 234–43
- McCracken P.J. et al. Proc. Intl. Soc. Mag. Reson. Med. 17 (2009) “Prostate Volume in Sexually Immature and Mature Dogs as Measured by MRI”
- Luo et al., 335:580, JPET 2010 “MRI Detection and Time Course of Cerebral Microhemorrhages during Passive Immunotherapy in Living APP Transgenic Mice”
- Hargreaves R. Imaging Substance P receptors (NK1) in the living human brain using positron emission tomography. J Clin Psychiatry 63 (suppl 11): 18-24, 2002
- Hargreaves, R. and Wagner, J. Imaging as a biomarker for decision making in drug development. In In Vivo MR Techniques in Drug Discovery and Development (ed. Beckmann, N.) Ch. 3, 31–46 (Taylor & Francis, New York, 2006),
- Rabiner E.A., Pharmacological differentiation of opioid receptor antagonists by molecular and functional imaging of target occupancy and food reward-related brain activation in humans. Molecular Psychiatry (2011) 16, 826–835
- Burns, H.D. et al. [18F]MK-9470, a positron emission tomography (PET) tracer or in vivo human PET brain imaging of the cannabinoid-1 receptor. Proc. Natl. Acad. Sci. USA 104, 9800–9805 (2007).
- Keller et al. Lack of efficacy of the Substance P (neurokinin1receptor) antagonist aprepitant in the treatment of major depressive disorder. BIOL PSYCHIATRY 59:216–223,2006.).
- Chawla et al. Establishing the dose of the oral NK1 antagonist aprepitant for the prevention of chemotherapy-induced nausea and vomiting. Cancer 97:2290-2300, 2003
- Warburg, O. On the origin of cancer cells. Science123, 309–314 (1956), Warburg et al. Über den Stoffwechsel der Carcinomzelle. Biochem. Zeitschr. 152, 309–344 1924
- Wahl et al, Metabolic monitoring of breast cancer chemohormonotherapy using positron emission tomography: initial evaluation. J Clin Oncol 11:2101-2111, 1993
- Weber, W.A. Use of PET for monitoring cancer therapy and predicting outcome. J Nucl Med 46:983-995, 2005.)
- Stroobants et al, 18FDG-positron emission tomography for the early prediction of response in advanced soft tissue sarcoma treated with imatinib mesylate (Gleevec). Eur J Cancer 39:2012-2020, 2003
- Prior et al. Early prediction of response to sunitinib after imatinib failure by 18F fluorodeoxyglucose positron emission tomography in patients with gastrointestinal stromal tumour. J Clin Oncol 27:439-445, 2009
- Benz et al. 18F-FDG PET/CT for monitoring treatment responses to the epidermal growth factor receptor inhibitor erlontinib. J Nucl Med 52:1684-1689, 2011
- Jacene et al. Assessment of interobserver reproducibility in quantitative 18F-FDG PET and CT measurements of tumour response to Therapy. J Nucl Med 50:1760-1769, 2009.)
- Von Hoff D.D., J Turner. Response rates, duration of response, and dose response effects in phase I studies of antineoplastics. InvestNew Drugs 9:115–22, 1991
- focr.org/12-15-2013-asco-post-oncology-drug-dosing-can-optimal-dose-be-fine-tuned-each-patient
- Tatiana Prowell, Nature Rev Drug Disc 13:410-411, 2014).
- Jain et al. Phase I oncology studies: Evidence that in the era of targeted therapies patients on lower doses do not fare worse. Clin Cancer Res 16:1289-1297, 2010.
- Shankar et al. Consensus recommendations for the use of 18F-FDG PET as an indicator of therapeutic response in patients in National Cancer Institute trials. J Nucl Med 47:1059- 1066, 2006
- Boellaard R. Need for standardization of 18F-FDG for PET-CT for treatment response assessments. J Nucl Med 52:93S-100S, 2011.).
Biography
Paul McCracken, PhD is Director of Imaging in the Biomarkers and Personalized Medicine Unit of Eisai Inc. Dr. McCracken joined Eisai in 2010 as Global Head of a new preclinical imaging group introducing PET, SPECT, MRI and CT to drug discovery, translation and development in oncology, neuroscience, and drug safety. Dr. McCracken holds a BS in Physics from The College of New Jersey, an MS in Physics/Medical Physics from Wright State University, and a PhD in Biomedical Engineering – Imaging from the Mayo Clinic College of Medicine. Prior to Eisai, Dr. McCracken was with Merck Research Laboratories, where he was part of a team responsible for development of preclinical and translational imaging biomarkers/strategies for assessment of efficacy and safety of newly developed molecular and biologic entities in several therapeutic areas.
Stephen Krause, PhD is a Principal Scientist in Imaging in the Biomarkers and Personalized Medicine Unit of Eisai Inc., joining in 2010. Dr. Krause received his PhD in Physiology at the Medical College of Virginia/Virginia Commonwealth University in 1983 and performed a post doc in Cardiology at Johns Hopkins Medical Institutions. Starting in 1986, Dr. Krause was an Assistant. Professor of Physiology at Thomas Jefferson University before joining Merck Research Laboratories in 1991 as a Scientist in Pharmacology and then Imaging.