Engineering cells and proteins – creating pharmaceuticals
Posted: 5 September 2014 | | No comments yet
Pharmaceutical biotechnology is big business; it currently consists of 1/6 of the total volume of the pharmaceutical market and continues to grow steadily. Expression of therapeutic proteins is mainly done in living cells, although ‘cell free protein synthesis’ (CFPS) or ‘in-vitro transcription translation’ (IVTT) is beginning to emerge as an alternative for commercial production. In this article we will highlight some of the more recent advances in protein expression systems for the production of pharmaceutical proteins. We will also discuss current trends in the engineering of pharmaceutical proteins with improved properties.
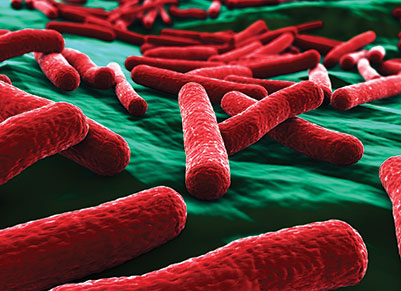
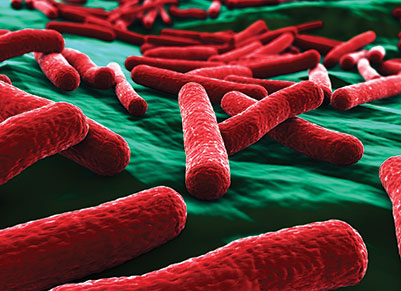
Pharmaceutical protein production
While proteins of interest are still isolated from natural extracts, the advent of modern molecular biology techniques has made recombinant protein expression the mainstream methodology for pharmaceutical production. The main drivers for the use of recombinant proteins are: low availability of native protein (e.g. the annual use of amylase and xylose isomerase is over 95,000 tons each); livestock infections for the production of vaccines and subsequent economic loss; immune responses to animal proteins after injection (e.g. insulin); and reproducibility of protein production in relation to its quality.
Since the implementation of recombinant DNA in the early-1970s, proteins have been expressed in many different organisms and (derived) cell types, such as bacteria, yeasts, moulds, insects, protozoa, mammals, plants, transgenic plants and animals and with the use of cell lysate in CFPS. During recombinant protein expression a gene is introduced in an organism (or its derivative) followed by constitutive- or induced-translation and transcription. Choosing the correct expression system is protein dependent and factors such as protein quality, functionality, production speed and yield are most important.
As of 2014, overall trends in recombinant protein production show an increase in the use of Chinese hamster ovary (CHO) and yeast cells, while there has been a decrease in the use of other mammalian and Escherichia coli (E. coli) systems. Production of non-glycosylated proteins is conducted mainly in bacteria or yeast (45%, May 2014), while the production of glycosylated proteins is carried out predominantly in CHO cells (29% compared to mammalian cells, insect cells and transgenic-animals and -plants at 26%. More than a decade ago about 20 pharmaceutical proteins were produced by transgenic technology for clinical trials14, however the high developmental costs for these production systems clearly hindered the advancement of this method. Other emerging systems in the market include the use of P. pastoris and H. polymorpha – both of which are discussed hereafter.
Escherichia coli (E.coli)
During the 1980s, E. coli was the dominating organism of choice for recombinant protein production15, and E. coli derived pharmaceutical proteins still account for 29% of marketed biologics. Due to the long history of its use and deep understanding of the E. coli genetics, much progress has been made in the engineering of E. coli strains for the production of proteins an plasmids10,12,16-19.
E. coli is a good choice for the first effort to produce a recombinant protein15, and a consensus protocol has been developed recently as a guide to start E. coli protein expression20. It can be cultivated with a relatively cheap defined media (e.g. glucose, ammonia phosphate and some minerals) and strategies for low cost production have been developed15,21. E. coli has clear advantages; however drawbacks include the incorrect formation of multiple disulfide bonds, non-extracellular production, and limited PTMs (e.g. glycosylation is not possible). Moreover, E. coli produces pyrogenic endotoxins, although various methods can be employed for their removal22. Typical protein yields are in the range of 20-400 mg/L medium (see Figure 2).
Yeast
Saccharomyces cerevesiae (S. cerevesiae), Pichia pastoris (P. pastoris) and Hansenula polymorpha (H. polymorpha) are currently the only three expression strains used in the production of marketed pharmaceutical proteins (see Figure 1). Yeasts are single-celled eukaryotic fungal organisms, and the major advantages of its use are: high yield (see Figure 2); stable production strains; durability; cost effective; high density growth; high productivity; suitability for production of isotopically-labelled protein; rapid growth in chemically defined media; product processing similar to mammalian cells; can handle S–S rich proteins; can assist protein folding; and its ability to glycosylate proteins12. Proteins that do not fold correctly in E. coli or that require glycosylation for its function are often produced in yeast.
Like E. coli, S. cerevesiae has a comparatively well-characterised genome and since the organism has no pathogenic properties it is classified as GRAS (generally regarded as safe). In general, S. cerevesiae is good alternative to E. coli, however the complex glycosylation patterns are often unacceptable for mammalian proteins because the O-linked oligosaccharides contain only mannose whereas higher eukaryotic proteins have sialylated O-linked chains. Additionally, N-linked sites are over-glycosylated, high mannose type structures which can lead to immunological responses and rapid clearance rates12,23.
The methylotrophic yeast P. pastoris is an effective and versatile system for the expression of heterologous proteins24. Its growing popularity can be attributed to several factors: (i) easy accessibility to well-established molecular biology techniques developed for S. cerevesiae; (ii) its ability to express proteins at high levels (either intracellular or extracellular); (iii) performs PTMs (i.e. glycosylation, disulfide bond formation, and proteolytic processing); (iv) the expression system is available as a commercial kit; (v) tightly regulated promoter systems were developed (e.g. AOX1, up to 1000 fold up-regulation); and (vi) capable of enduring high cell density cultivations in a bioreactor and the preference of a respiratory- over a fermentative-growth model25,26. Glycosylation is less extensive in P. pastoris than in S. cerevisiae27. N-linked high-mannose oligosaccharides are usually up to 20 residues. Human-like hybrid and complex N-glycans were generated in P. pastoris28,29, and these systems are currently optimised30-32.
An in-depth review of H. polymorpha by Gotthard and co-workers highlights its strengths for pharmaceutical protein production33. In short, the generation of recombinant H. polymorpha strains typically employs vectors, traditionally circular plasmids, which are mitotically stable being integrated into the genome of the host. Integration over a number of generations may result in strains with as many as 100 integrated plasmids present in tandem repeats. A major advantage of H. polymorpha is that proteins can be secreted into the media, or into a pre-selected cell compartment, such as the peroxisome, the vacuole, or targeted to the cell surface. For secreted phytase, product levels of up to 13.5 g/L have been obtained.
Compared to S. cerevisiae, the high mannose glycan chains of N-linked oligosaccharides generally appear to be much shorter in H. polymorpha; typical oligosaccharide species attached to the recombinant protein have Man8-12 GlcNAc2-structures without terminal α-1,3-linked mannose residues. Therefore, the outer chain processing in the N-linked glycosylation pathway in H. polymorpha appears to be similar to that in P. pastoris, with the addition of shorter mannose structures and lack of any terminal α-1,3-linked mannose residues.
Mammalian cells
Today over 50% of all recombinant protein pharmaceuticals are produced in mammalian cells (see Figure 1). Driven by the need of PTMs of pharmaceutical proteins, these relatively complex expression systems have increased their yields tremendously due to developments in bioprocess engineering, media optimisation, and strain engineering since the 1980s34 . While adherent cell cultures are used in industrial setting, suspended cell cultures (e.g. CHO cell- and BKH cell-cultures), and for even more increased yields, extended batch cultures and perfusion processes in phase III- or the production-phase are most abundant.
While biopharmaceuticals requiring relatively little or no PTM’s can be made in platforms ranging from E. coli to mammalian cells, glycoproteins and other pharmaceuticals requiring more complex PTM’s, e.g. glycosylation, mammalian cell lines are the only viable option. This is because the final glycosylation pattern of recombinant proteins is determined by the expression platform; it is well known that there are significant differences in glycan structures between proteins expressed in human, mammalian and yeast cells (see Figure 3). As such, the majority of therapeutic glycoproteins are expressed in mammalian cell lines (e.g. CHO cells)35.
However, the drawbacks to mammalian expression include the number of glycoforms that are expressed and the differences in protein glycosylation between different mammalian cell lines36. For example, glycoproteins expressed in some cell lines, including CHO cells, contain terminal Neu5Gc (N-glycolylneuraminic acid) rather than the human Neu5Ac (N-acetylneuraminic acid). These non-human sialic acids moieties may affect immunogenicity as antibodies against the Neu5Gc have been identified35. Expression in some cell lines, e.g. epoetin delta in human fibrosarcoma cell line HT-1080, can result in glycan chains with no terminal Neu5Gc residues37, however this method is not always a possibility. One way to circumvent this problem, as well as other issues with PTM such as disulfide bond formation is to engineer cell lines to express proteins with the correct PTMs38.
Future outlook
While transient gene expression in mammalian cells is a maturing technology it is not yet approved for pharmaceutical protein production39. Engineering mammalian gene switches and post-transcriptional control (e.g. via RNA aptamer-intramer fusions) will give light to new expression strains: (i) as part of autologous cell therapies, gene circuits encode computational operations that can be programmed by intracellular signals to execute specific tasks, (ii) cell implants consisting of engineered allogeneic or xenogeneic mammalian cells could be plugged into the metabolism of patients to sense and respond to specific biomarkers40.
In addition, the trypanosomatid protozoa Leishmania tarentolae (a nonpathogenic parasite) is under investigations as an alternative production-system for pharmaceutical proteins due to its complex PTMs and significantly easier cultivation requirements than mammalians cells41-45.
Finally, with the first successful commercial production of antibodies in CFPS for the pharmaceutical industry by Sutro Biopharma, CA, USA in a 100 L tank6,7,46 the road is open to start producing more pharmaceutical proteins in not only E. coli lysates, but also eukaryote cell lysates.
Engineering of biopharmaceuticals
Protein engineering can span a wide field of modifications and conjugations including gene manipulations, glycosylation, PEGylation, albumin fusion, PASylation, fatty acid conjugation, amidation, disulfide bond shuffling, and Fc fusion. Here focus on protein engineering methods applied post-expression for improved stability, solubility, potency, reduced immunogenicity, increased proteolytic resistance, and/or improved serum half-life.
Glycoengineering
N-linked glycosylation is known to mediate a wide spectrum of functions; the most important of which (in terms of protein pharmacokinetics) include activity, stability, solubility, folding, immunogenicity and in-vivo half-life. As such, pharmaceutical glycoproteins that are expressed non-glycosylated (i.e. in E. coli) can exhibit impaired in-vivo activities and in some cases complete loss of in-vivo activity is observed. Recombinant human interferon-β (rhIFN-β) is an example of a naturally glycosylated protein that is active in-vivo when expressed from E. coli (i.e. non-glycosylated)47. However, while both the glycosylated and non-glycosylated variant is available as a biopharmaceutical, the solubility and activity of the non-glycosylated variant is impaired in relation to its glycosylated counterpart. This example highlights the importance of glycosylation in the context of pharmaceutical proteins and the ability to control glycosylation is continually progressing.
Approaches to glycoengineering can vary widely. The most highly cited example of successful glycoengineering is darbepoietin alfa, the long acting erythropoietin derivative that mediates red blood cell production for the treatment of anemia in association with chronic kidney disease (CKD) and/or chemotherapy treatment48,49. Epogen/ Procrit was the first erythropoiesis-stimulating agent (ESA) to be approved in 1989 and the active ingredient, epoetin alfa, is recombinant human erythropoietin expressed in CHO cells. Darbepoietin alfa, marketed as Aranesp by Amgen in 2001, has two additional N-linked glycosylation sites engineered into the peptide backbone. The resulting increase in sialic acid content gives Aranesp a lower affinity for the EPO receptor (Aranesp IC50 of 1.05 compared to epoetin alfa IC50 of 0.54 ng) but an increased serum half-life (Aranesp has a t1/2 of 26 hours compared to 8.5 hours for epoetin alfa)50. As a result, Aranesp can be administered less frequently than epoetin alfa products.
While Aranesp is the only approved drug utilising this methodology, recent examples of ‘hyperglycosylated’ proteins with an improved half-life include coagulation factor IX (four additional glycosylation sites were introduced to yield a variant with a 2.4 fold increase in half-life)51, recombinant human IFN-alpha2 (4- and 5-N-linked IFN variants showed a 25-fold increase in half-life compared to the non-glycosylated hIFN-alpha2)52, human alpha 1-antitrypsin, A1AT (one additional glycosylation site at various positions yielded variants with up to a 3.6-fold longer serum half-life)53, and follicle-stimulating hormone, FSH, (introduction of 4 additional N-linked glycosylation sites yielded a variant with a 2-fold increase in half-life)54. Interestingly, in the case of the FSH, both N- and O-linked glycosylation sites were introduced into the protein. While the N-linked variants showed a significantly increased half-life compared to the O-linked variants, it highlights the use of a relatively less understood and underexploited form of PTM54. Other approaches to glycoengineering include the generation of afucosylated glycoproteins and antibodies, enzymatic cleavage/ addition of glycans and glycoPEGylation55-60.
PEGylation
PEGylation is by far the most prominent conjugation strategy currently used to improve the pharmacokinetics of therapeutic proteins. PEGylation was originally introduced as a method to prevent immune responses in patients; however since then it has emerged as a versatile tool to increase solubility, reduce toxicity and prevent protein aggregation.
The currently available PEGylated biopharmaceuticals span a range of therapeutics with modifications ranging from the addition of a single PEG moiety up to the addition of 9 PEG moieties (see Table 1)61-74. Despite the success of PEGylated proteins in the market, a shortcoming to PEGylation technology is the limited control over the site of ligation; PEG is most often ligated to proteins via accessible amino groups, either the N-terminus or surface lysine residues. Approaches to achieve site specific conjugation revolve around the exploitation of naturally occurring ‘handles’ for ligation such as the modification/introduction of a ‘free’ cysteine75-78 and the use of naturally occurring glycosylation sites, e.g. glycoPEGylation.
In some cases PEGylation can result in lower in-vitro activities as the modification can be within a receptor binding region or active site; however, this loss of in-vitro activity is usually compensated for by an increase in in-vivo activity as a result of increased circulating half-life79-83. This is the case for Mircera, a PEGylated epoetin beta that acts as a continuous erythropoietin receptor activator80. After expression in CHO cells, epoetin beta is chemically modified with a single 30kDa PEG. This modification results in a lower affinity for the EPOreceptor but an increased half-life (t1/2 of 134 hours compared to 8.5 hours for epoetin alfa and 26 hours for Aranesp)82.
GlycoPEGylation, successfully applied to Lonquex (lipegfilgrastim), is the combination of a glycoengineering and PEGylation technology. Lonquex is granulocyte colony stimulating factor (G-CSF) which has been enzymatically modified with a 20-kDa PEG-sialic acid derivative. To achieve this, G-CSF is expressed in E. coli and the 20-kDa PEG-sialic acid derivative is transferred to the unused natural O-linked glycosylation site with a truncated N-acetylgalactosaminyltransferase isoform64. This approach has also been applied to, interferon-alpha2b (IFN-α2b), granulocyte/macrophage colony stimulating factor (GM-CSF), and recombinant activated factors VII, VIII and IX84-87.
Incorporation of unnatural amino acids
Another emerging field in protein engineering for the site-specific conjugation of functional moieties to biopharmaceuticals is the incorporation of unnatural amino acids (uAA). The expansion of the genetic code is essentially the recoding of an antisense codon to biosynthetically incorporate unnatural amino acids into protein scaffolds88,89. This work was first pioneered in an E.coli system by Shultz and co-workers, where the specificity of the Methanococcus jannaschii tyrosyl tRNA synthetase was redirected towards uAAs88,89.
The essential components for the incorporation of uAA’s are an aminoacyl tRNA synthetase (aaRS) that charges a specific tRNA with a uAA and an orthogonal tRNA anticodon mutated to recognise a nonsense codon e.g. the amber stop codon TAG. The aaRS-tRNA must be orthogonal with respect to the expression system – the aaRS must not recognise any host cell tRNA and the tRNA must not be aminoacylated by any host aaRS.
This approach has been utilised in E. coli-, mammalian-, yeast-cells and cell free platforms87-97. Current restrictions associated with uAA incorporation include low overall product yield, the efficiency of the uAA incorporation and the subsequent conjugation efficiency. Despite this, there have been a number of successful examples of conjugated pharmaceutical proteins developed using this methodology, especially for the generation of antibody-drug conjugates93,95-100. The pharmaceutical company Ambrx has recently published the production of Antibody Drug Conjugates in CHO cells using uAA incorporation via their EuCODE technology86. Expression yields for this system were on the 1g/L scale with an overall conjugation yield of 95%.
Concluding remarks
Over the last 20 years more complex expression systems have come into use. With rapid developments in post-translational engineering and strain development to produce even better defined products, faster approval of biopharmaceuticals can be expected, especially if transient cell expression and CFPS production methods are approved. After the revolution of modern molecular biology to the field of biopharmaceuticals it is time for a PTM revolution via synthetic biology and chemical conjugations.
References
- Walsh G.Biopharmaceutical benchmarks. Nature Biotechnology, 2003, 21, 865-870
- Walsh G. Biopharmaceutical benchmarks. Nature Biotechnology, 2010, 28, 917-924
- Walsh G. A review of new biologic drug approvals over the years, featuring highlights from 2010 and 2011. Biopharm International, 2012, 6, 34-36
- Walsh G. Milestones and moderate progress in 2012 drug approvals. Biopharm International, 2013, 26, 54-56
- Walsh G. Biopharmaceutical approval trends in 2013. Biopharm International, 2014 (online May 27th, 2014: http://www.biopharminternational.com/biopharm/Business/Biopharmaceuticals-Approval-Trends-in-2013/ArticleStandard/Article/detail/839471)
- Swartz JR. Transforming biochemical engineering with cell-free biology. AIChE Journal, 2012, 58, 5–13
- Casteleijn MG, Urtti A, Sarkhel S Expression without boundaries: Cell-free protein synthesis in pharmaceutical research International Journal of Pharmaceutics, 2013, 440, 39-47
- Lutz S, Bornscheuer UT Protein Engineering Handbook 2008, (1st ed)Wiley-VCH, Weinheim
- Rader RA. FDA Biopharmaceutical Product Approvals and Trends in 2012; Up from 2011, but Innovation and Impact Are Limited. BioProcess International, 2013, 11, 18-27
- Hunt I. From gene to protein: a review of new and enabling technologies for multi-parallel protein expression. Protein Expression and Purification, 2005, 40, 1-22
- Schmidt FR. From Gene to Product: The Advantage of Integrative Biotechnology. Gad SC (Editor) Handbook of Pharmaceutical Biotechnology, 2007, 2-38
- Demain AL, Vaishnav P Production of recombinant proteins by microbes and higher organisms Biotechnology Advances, 27, 2009, 297-306
- Jayaraj R, Smooker PM. So you Need a Protein – A Guide to the Production of Recombinant Proteins. The Open Veterinary Science Journal, 2009, 3, 28-34
- Walsh G. Biopharmaceutical benchmarks. Nature Biotechnology, 2000, 18, 831-8333.
- Enfors SO, Häggström L. Bioprocess Technology Fundamentals and Applications (1st edition). Högskoletryckeriet, Royal Insitute of Technology (KTH), Stockholm (2000)
- Chou CP. Engineering cell physiology to enhance recombinant protein production in Escherichia coli. Applied Microbioly and Biotechnology, 2007, 76, 521–532
- Samuelson JC. Recent Developments in Difficult Protein Expression: A Guide to E coli Strains, Promoters, and Relevant Host Mutations. Thomas CE Jr, Ming-Qun X (Editors). Heterologous Gene Expression in E coli: Heterologous Gene Expression in Ecoli, Methods in Molecular Biology, 2011, 705, 195-209
- Baneyx Recombinant protein expression in Escherichia coli. Current Opinion in Biotechnology, 1999, 10, 5, 411–421
- Casali N. Escherichia coli Host Strains. Preston A (Editor) E coli Plasmid Vectors: Methods in Molecular Biology™, 2003, 235, 27-48
- Gräslund S, Nordlund P, Weigelt J, Hallberg BM, Bray J, Gileadi O, Knapp S, Oppermann U, Arrowsmith C, Hui R, Ming J, dhe-Paganon S, Park H-W, Savchenko A, Yee A, Edwards A, Vincentelli R, Cambillau C, Kim R, Kim S-H, Rao Z, Shi Y, Terwilliger TC, Kim C-Y, Hung L-W, Waldo GS, Peleg Y, Albeck S, Unger T, Dym O, Prilusky J, Sussman JL, Stevens RC, Lesley SA, Wilson IA, Joachimiak A, Collart F, Dementieva I, Donnelly MI, Eschenfeldt WH, Kim Y, Stols L, Wu R, Zhou M, Burley SK, Emtage JS, Sauder JM, Thompson D, Bain K, Luz J, Gheyi T, Zhang F, Atwell S, Almo SC, Bonanno JB, Fiser A, Swaminathan S, Studier FW, Chance MR, Sali A, Acton TB, Xiao R, Zhao L, Ma LC, Hunt JF, Tong L, Cunningham K, Inouye M, Anderson S, Janjua H, Shastry R, Ho CK, Wang D, Wang H, Jiang M, Montelione GT, Stuart DI, Owens RJ, Daenke S, Schütz A, Heinemann U, Yokoyama S, Büssow K, Gunsalus KC. Protein production and purification. Nature Methods, 2008, 5, 135-146
- Peti W, Page R. Strategies to maximize heterologous protein expression in Escherichia coli with minimal cost. Protein Expression and Purification, 2007, 51 1–10
- Petsch D, Anspach FB. Endotoxin removal from protein solutions. Journal of Biotechnology, 2000, 76, 97-119
- Nasaba FP, Aebia M, Bernhardb G, Frey AD. A Combined System for Engineering Glycosylation Efficiency and Glycan Structure in Saccharomyces cerevisiae. Applied and Environmental Microbiology, 2013, 79, 997-1007
- Potvin G. Ahmad A. Zhang Z. Bioprocess engineering aspects of heterologous protein production in Pichia pastoris: A review. Biochemical Engineering Journal, 2012, 64, 91-105
- Cereghino GP, Cereghino JL, Ilgen C, Cregg JM. Production of recombinant proteins in fermenter cultures of the yeast Pichia pastoris. Current Opinion in Biotechnology, 2002, 13, 329-332
- Cereghino JL, Cregg JM. Heterologous protein expression in the methylotrophic yeast Pichia pastoris. FEMS Microbiology Reviews, 200, 24, 45–66
- Dale C, Allen A, Fogarty S. Pichia pastoris: a eukaryotic system for the large-scale production of biopharmaceuticals. Biopharm, 1999, 12, 36–42
- Choi BK, Bobrowicz P, Davidson RC, Hamilton SR, Kung DH, Li H, Miele RG, Nett JH, Wildt S, Gerngross TU. 2003. Use of combinatorial genetic libraries to humanize N-linked glycosylation in the yeast Pichia pastoris. PNAS, 2003,100, 5022–5027
- Hamilton SR, Bobrowicz P, Bobrowicz B, Davidson RC, Li H, Mitchell T, Nett JH, Rausch S, Stadheim TA, Wischnewski H, Wildt S, Gerngross TU. Production of complex human glycoproteins in yeast. Science, 2003,301, 1244–1246
- Vervecken W, Kaigorodov V, Callewaert N, Geysens S, De Vusser K, Contreras R. In vivo synthesis of mammalian-like, hybrid-type N-glycans in Pichia pastoris. Applied and Environmental Microbiology, 2004, 70, 2639–2646
- Bobrowicz P, Davidson RC, Li H, Potgieter TI, Nett JH, Hamilton SR, Stadheim TA, Miele RG, Bobrowicz B, Mitchell T, Rausch S, Renfer E, Wildt S. Engineering of an artificial glycosylation pathway blocked in core oligosaccharide assembly in the yeast Pichia pastoris: production of complex humanized glycoproteins with terminal galactose. Glycobiology, 200414,757–766
- Hamilton SR, Davidson RC, Sethuraman N, Nett JH, Jiang Y, Rios S, Bobrowicz P, Stadheim TA, Li H, Choi BK, Hopkins D, Wischnewski H, Roser J, Mitchell T, Strawbridge RR, Hoopes J, Wildt S, Gerngross TU. 2006. Humanization of yeast to produce complex terminally sialylated glycoproteins. Science313:1441–1443
- Gotthard K, Kang HA, Gellissen G. Hansenula polymorpha (Pichia angusta): Biology and Applications. Satyanarayana T, Gotthard K (Editors). Yeast Biotechnology: Diversity and Applications, 2009, 47-64
- Wurm FM. Production of recombinant protein therapeutics in cultivated mammalian cells. Nature Biotechnology, 2004, 22, 1393 – 1398
- Ghaderi D, Zhang M, Hurtado-Ziola N, Varki A. Production platforms for biotherapeutic glycoproteins. Occurrence, impact, and challenges of non-human sialylation. Biotechnology & genetic engineering reviews, 2012, 28, 147-75.
- Croset A, Delafosse L, Gaudry J-P, Arod C, Glez L, Losberger, C, Begue D, Krstanovic A, Robert F, Vilbois F, Chevalet L, Antonsson B, Differences in the glycosylation of recombinant proteins expressed in HEK and CHO cells, Journal of Biotechnology, 2012, 161, 336-348
- Llop E, Gutiérrez-Gallego R, Segura J, Mallorquí J, Pascual JA. Structural analysis of the glycosylation of gene-activated erythropoietin (epoetin delta, Dynepo). Analytical Biochemistry, 2008, 383, 243-254
- Lai T, Yang Y, Ng SK. Advances in Mammalian Cell Line Development Technologies for Recombinant Protein Production. Pharmaceuticals, 2013, 6, 579-603.
- De Jesus MJ, Wurm FM. Mammalian Cells in Biotech Production. Kayser O, Warzecha H (Editors). Pharmaceutical Biotechnology: Drug Discovery and Clinical Applications, Second Edition, 2012, 43-57
- Ausländer S, Fussenegger M. From gene switches to mammalian designer cells: present and future prospects. Trends in Biotechnology, 2013, 31, 155–168
- Basile G, Peticca M. Recombinant Protein Expression in Leishmania tarentolae. Molecular Biotechnology, 2009, 43, 273-278
- Jørgensen ML, Friis NA, Just J, Madsen P, Petersen SV, Kristensen P. Expression of single-chain variable fragments fused with the Fc-region of rabbit IgG in Leishmania tarentolae. Microbial Cell Factories, 2014, 13:9
- Sepúlveda S, Valenzuela L, Ponce I, Sierra S, Bahamondes P, Ramirez S, Rojas V, Kemmerling U, Galanti N, Cabrera G. Expression, functionality, and localization of apurinic/apyrimidinic endonucleases in replicative and non-replicative forms of Trypanosoma cruzi. Journal of Cellular Biochemistry, 2014, 115: 397-409
- Taromchi AH, Kazemi B, Mahmazi S, Bandehpour M. Heterologous Expression of Human IL-29 (IFN-λ1) in Iranian Lizard Iranian Journal of Biotechnology, 2013, 11, 168-174
- Niimi Recombinant Protein Production in the Eukaryotic Protozoan Parasite Leishmania tarentolae: A Review. Methods in Molecular Biology, 2012, 824, 307-315
- Zawada JF, Yin G, Steiner AR, Yang J, Naresh A, Roy SM, DS Gold DS, Heinsohn HG, Murray CJ Microscale to manufacturing scale-up of cell-free cytokine production – a new approach for shortening protein production development timelines Biotechnology and Bioengineering, 2011, 108, 1570 – 1578
- Runkel L, Meier W, Pepinsky R, Karpusas M, Whitty A, Kimball K, Brickelmaier M, Muldowney C, Jones W, Goelz S,. Structural and functional differences between glycosylated and non-glycosylated forms of human interferon-beta (IFN-beta). Pharmaceutical research, 1998, 15, 641-649
- Data from www.ema.europa.eu and www.fda.gov
- Debeljak N, Sytkowski A,. Erythropoietin and erythropoiesis stimulating agents. Drug Testing and Analysis, 2012, 4, 1942-7611
- Egrie J, Browne J,. Development and characterization of novel erythropoiesis stimulating protein (NESP). 2011, British journal of cancer, 3-10
- Bolt G, Bjelke J, Hermit M, Hansen L, Karpf D, Kristensen C,. Hyperglycosylation prolongs the circulation of coagulation factor IX. Journal of thrombosis and haemostasis : 2012, 10, 2397-2398
- Ceaglio N, Etcheverrigaray M, Kratje R, and Oggero M,. Novel long-lasting interferon alpha derivatives designed by glycoengineering. Biochimie, 2008, 90, 437-449
- Lusch A, Kaup M, Marx U, Tauber R, Blanchard V, Berger M. Development and analysis of alpha 1-antitrypsin neoglycoproteins: the impact of additional N-glycosylation sites on serum half-life. Molecular pharmaceutics, 2013, 10, 2616-2629
- Weenen C, Peña J, Pollak S, Klein J, Lobel L, Trousdale R, Palmer S, Lustbader E, Ogden R, Lustbader J,. Long-acting follicle-stimulating hormone analogs containing N-linked glycosylation exhibited increased bioactivity compared with o-linked analogs in female rats. The Journal of clinical endocrinology and metabolism, 2004, 89, 5204-5212
- Jefferis R. Glycosylation as a strategy to improve antibody-based therapeutics. Nature Reviews Drug Discovery, 2009, 8, 226-234
- Solá R, Griebenow K,. Effects of glycosylation on the stability of protein pharmaceuticals. Journal of pharmaceutical sciences, 2009, 98, 1223-1245
- Satoh M , Iida S, Shitara K,. Non-fucosylated therapeutic antibodies as next-generation therapeutic antibodies. 2006, 6, 1161-1173
- Golay J, Da Roit F, Bologna L, Ferrara C, Leusen J, Rambaldi A, Klein C, Introna M. Glycoengineered CD20 antibody obinutuzumab activates neutrophils and mediates phagocytosis through CD16B more efficiently than rituximab. Blood, 2013, 122, 3482-3491
- Zhou Q, Stefano J, Manning C, Kyazike J, Chen B, Gianolio D, Park A, Busch M, Bird J, Zheng X, Simonds-Mannes H, Kim J, Gregory R, Miller R, Brondyk W, Dhal P, Pan C,. Site-specific antibody-drug conjugation through glycoengineering. Bioconjugate chemistry, 2014, 25, 510-520
- Walsh G, Jefferis R. Post-translational modifications in the context of therapeutic proteins. Nature biotechnology, 2006, 24, 1241-1252
- Curran MP, McCormack PL. Methoxy Polyethylene Glycol-Epoetin Beta. Drugs, 2008, 68, 1139 – 1156
- Alconcel SNS, Baas AS, Maynard HD. FDA-approved poly(ethylene glycol)–protein conjugate drugs. Polymer Chemistry, 2011, 2, 1442-1448
- Stead RB, Lambert J, Wessels D, Iwashita JS, Leuther KK, Woodburn KW, Schatz PJ, Okamoto DM, Naso R, Duliege AM. Evaluation of the safety and pharmacodynamics of Hematide, a novel erythropoietic agent, in a phase 1, double-blind, placebo-controlled, dose-escalation study in healthy volunteers. Blood, 2006,108, 1830-1834
- Mahlert F, Schmidt K, Allgaier H, Liu SP. Rational Development Of Lipegfilgrastim, a Novel Long-Acting Granulocyte Colony-Stimulating Factor, Using Glycopegylation Technology. Blood, 2013, 122, 4853
- Sherman MR, Saifer MGP, Perez-Ruiz F. PEG-uricase in the management of treatment-resistant gout and hyperuricemia.Advanced Drug Delivery Reviews, 2008, 60, 59-68
- Sundy JS, Ganson NJ, Kelly, SJ, Scarlett EL, Rehrig CD, Huang W, Hershfield MS. Pharmacokinetics and pharmacodynamics of intravenous PEGylated recombinant mammalian urate oxidase in patients with refractory gout. Arthritis & Rheumatism, 2007, 56, 1021-1028
- Kling J. PEGylation of Biologics. BioProcess International, 2013, 11, 34–43
- Möller I, Thomas A, Geyer H, Schänzer W, Thevis M. Synthesis, characterisation, and mass spectrometric detection of a pegylated EPO‐mimetic peptide for sports drug testing purposes.
- Wrighton NC, Farrell FX, Chang R, Kashyap AK, Barbone FP, Mulcahy LS, Johnson DL, Barrett RW, Jolliffe LK, Dower WJ. Small peptides as potent mimetics of the protein hormone erythropoietin. Science, 1996, 273, 458-64.
- Ng, EWM, Shima DT, Calias P, Emmett T. Cunningham-Jr ET, Guyer DR. Adamis AP. Pegaptanib, a targeted anti-VEGF aptamer for ocular vascular disease. Nature Reviews Drug Discovery 5, 123-132
- Simona J, Menci K, Vladka GP. PEGylation of therapeutic proteins. Biotechnology Journal, 2010, 5, 113–128
- Pradhananga S, Wilkinson I, Ross RJM. Pegvisomant: structure and function. Journal of Molecular Endocrinology, 2002, 29, 11-14
- Crawford J. Pegfilgrastim: the promise of pegylation fulfilled. Annals of Oncology, 2003, 14, 6-7
- Lai L, Hui CK, Leung N, Lau GK. Pegylated interferon alpha-2a (40 kDa) in the treatment of chronic hepatitis B. International Journal of Nanomedicine, 2006, 1, 255–262
- Jevševar S, Kunstelj M, Porekar V. PEGylation of therapeutic proteins. Biotechnology Journal, 2010, 5
- See bolderbio.com
- See creativepegworks.com
- Cong Y, Pawlisz E, Bryant P, Balan S, Laurine E, Tommasi R, Singh R, Dubey S, Peciak K, Bird M, Sivasankar A, Swierkosz J, Muroni M, Heidelberger S, Farys M, Khayrzad F, Edwards J, Badescu G, Hodgson I, Heise C, Somavarapu S, Liddell J, Powell K, Zloh M, Choi J, Godwin A, Brocchini S,. Site-specific PEGylation at histidine tags. Bioconjugate chemistry, 2012, 23, 248-263
- Harris J, Chess R. Effect of PEGylation on pharmaceuticals. Nature reviews. Drug discovery, 2003, 214-221
- Fishburn C. The pharmacology of PEGylation: balancing PD with PK to generate novel therapeutics. Journal of pharmaceutical sciences, 2008, 97, 4167-4183
- Kling J. PEGylation of Biologics. BioProcess International, 2013, 11, 34–43
- Curran M, McCormack P. Methoxy polyethylene glycol-epoetin beta: a review of its use in the management of anaemia associated with chronic kidney disease. Drugs, 2008, 68, 1139-1156
- Alconcel S, Bass A, and Maynard FDA-approved poly(ethylene glycol)–protein conjugate drugs. Polymer chemistry, 2011, 2, 1442-1448
- Golor G, Bensen-Kennedy D, Haffner S, Easton R, Jung K, Moises T, Lawo J-P, Joch C, Veldman A,. Safety and pharmacokinetics of a recombinant fusion protein linking coagulation factor VIIa with albumin in healthy volunteers. Journal of thrombosis and haemostasis, 2013, 11, 1977-1985
- Negrier C, Knobe K, Tiede A, Giangrande P, Møss J,. Enhanced pharmacokinetic properties of a glycoPEGylated recombinant factor IX: a first human dose trial in patients with hemophilia B. , 2011, 118, 2695-2701
- Stennicke H, Kjalke M, Karpf D, Balling K, Johansen P, Elm T, Øvlisen K, Möller F, Holmberg H, Gudme C, Persson E, Hilden I, Pelzer H, Rahbek-Nielsen H, Jespersgaard C, Bogsnes A, Pedersen A, Kristensen A, Peschke B, Kappers W, Rode F, Thim L, Tranholm M, Ezban M, Olsen E, Bjørn S,. A novel B-domain O-glycoPEGylated FVIII (N8-GP) demonstrates full efficacy and prolonged effect in hemophilic mice models. Blood, 2013, 121, 2108-2116
- DeFrees S, Wang Z, Xing R, Scott AE, Wang J, Zopf D, Gouty DL, Sjoberg ER, Panneerselvam K, Brinkman-Van der Linden ECM, Bayer RJ, Tarp MA, Clausen H,. GlycoPEGylation of recombinant therapeutic proteins produced in Escherichia coli. Glycobiology, 2006, 16, 833-843
- Wang L, Brock A, Herberich B, Schultz P,. Expanding the genetic code of Escherichia coli. Science, 2001, 292, 498-500
- Young T, Schultz P. Beyond the canonical 20 amino acids: expanding the genetic lexicon. The Journal of biological chemistry, 2010, 285, 11039-11044
- Chin J, Cropp T, Anderson J, Mukherji M, Zhang Z, Schultz P,. An expanded eukaryotic genetic code. Science, 2003, 301, 964-967
- Sakamoto K, Hayashi A, Sakamoto A, Kiga D, Nakayama H, Soma A, Kobayashi T, Kitabatake M, Takio K, Saito K, Shirouzu M, Hirao I, Yokoyama S,. Site-specific incorporation of an unnatural amino acid into proteins in mammalian cells. Nucleic acids research, 2002, 30, 4692-4699
- Kowal A, Kohrer C, RajBhandary U,. Twenty-first aminoacyl-tRNA synthetase-suppressor tRNA pairs for possible use in site-specific incorporation of amino acid analogues into proteins in eukaryotes and in eubacteria. Proceedings of the National Academy of Sciences of the United States of America, 2001, 2268-2273
- Zimmerman E, Heibeck T, Gill A, Li X, Murray C, Madlansacay M, Tran C, Uter N, Yin G, Rivers P, Yam A, Wang W, Steiner A, Bajad S, Penta K, Yang W, Hallam T, Thanos C, Sato A. Production of site-specific antibody-drug conjugates using optimized non-natural amino acids in a cell-free expression system. Bioconjugate chemistry , 2014, 25, 351-361
- Goerke A, Swartz J. High-level cell-free synthesis yields of proteins containing site-specific non-natural amino acids. Biotechnology and bioengineering, 2009, 400-416
- Ozawa K, Loscha K, Kuppan K, Loh C, Dixon N, Otting G,. High-yield cell-free protein synthesis for site-specific incorporation of unnatural amino acids at two sites. Biochemical and biophysical research communications, 2012, 418, 652-656
- Jackson D, Atkinson J, Guevara C, Zhang C, Kery V, Moon S, Virata C, Yang P, Lowe C, Pinkstaff J, Cho H, Knudsen N, Manibusan A, Tian F, Sun Y, Lu Y, Sellers A, Jia X, Joseph I, Anand B, Morrison K, Pereira D, Stover D. In vitro and in vivo evaluation of cysteine and site specific conjugated herceptin antibody-drug conjugates. PloS one, 2014, 9, 34-45
- Panowksi S, Bhakta S, Raab H, Polakis P, Junutula J. Site-specific antibody drug conjugates for cancer therapy. mAbs, 2014, 6, 34-45
- See allozyne.com
- See ambrx.com
- Tian F, Lu Y, Manibusan A, Sellers A, Tran H, Sun Y, Puong T, Barnett R, Hehli B, Song F, DeGuzman M, Ensari S, Pinkstaff J, Sullivan L, Biroc S, Cho H, Schultz P, DiJoseph J, Dougher M, Ma D, Dushin R, Leal M, Tchistiakova L, Feyfant E, Gerber H, Sapra P. A general approach to site-specific antibody drug conjugates. Proceedings of the National Academy of Sciences of the United States of America, 2014, 111, 1766-1771
Biography
Marco Casteleijn is working at the Centre for Drug Research at the Faculty of Pharmacy of the University of Helsinki in Finland. He obtained his Ba in Life Sciences in Utrecht, the Netherlands. Before his PhD, Marco was Test Supervisor and Researcher at the National Institute for Public Health and the Environment at the Dutch drug regulatory offices for biological drug release, and worked as a technician for a fresh water provider (microbiology and analytical chemistry) and in the cosmetics and food industry. Marco obtained his Msc in Biochemistry and his PhD in Bioprocess Engineering at the University of Oulu in Finland where he worked in protein engineering projects on industrial enzymes. He is currently working on pharmaceutical proteins: development and delivery. He has published several peer review articles on protein engineering, bioprocess development, and industrial enzymes. In addition, Marco has a background in quality assurance, was a board member of the Biocenter Oulu graduate school, and is on the scientific advisory board of a German Biotech company. Email: [email protected].
Dominique Richardson graduated with a Master of Chemistry from the University of Durham in 2009. She subsequently undertook her PhD studies in protein biochemistry at the University of Manchester with Prof. Sabine Flitsch. Upon completing this in 2013, she joined the University of Helsinki where she currently works as a postdoctoral researcher with Prof. Arto Urtti on the development of a ‘capture and release’ cell-free platform for the expression and screening of pharmaceutical proteins.