In vivo drug target validation using RNAi
Posted: 21 July 2007 | | No comments yet
Among the genetic model organisms, the laboratory mouse (Mus musculus) has a predominant role in the study of human disease and in pre-clinical drug development. Apart from the high degree of sequence homology of mouse and human genomes, and similarities in many physiological aspects, advanced targeting technologies make the crucial difference; providing unique tools for elucidating gene function in vivo.
Among the genetic model organisms, the laboratory mouse (Mus musculus) has a predominant role in the study of human disease and in pre-clinical drug development. Apart from the high degree of sequence homology of mouse and human genomes, and similarities in many physiological aspects, advanced targeting technologies make the crucial difference; providing unique tools for elucidating gene function in vivo.
Among the genetic model organisms, the laboratory mouse (Mus musculus) has a predominant role in the study of human disease and in pre-clinical drug development. Apart from the high degree of sequence homology of mouse and human genomes, and similarities in many physiological aspects, advanced targeting technologies make the crucial difference; providing unique tools for elucidating gene function in vivo.
The ability to manipulate the genome in ES cells and mice was developed in the late 1980s; since then, gene targeting has been used extensively to study gene function in genetically modified mouse strains. As initially designed; the technique allows the disruption of a target gene in the murine germline by the insertion of a selectable marker. About 4.000 “knock-out” (KO) mice have been described in the literature, demonstrating the wide use of this approach. The application of Cre/loxP recombination has refined the tools for manipulating the mouse genome; detecting site and timing of gene alteration in the living animal1-3. An inherent feature of these recombinase-based approaches, however, is a non-reversible gene switch that does not allow modulating gene expression in a given cell. In addition, the derivation of conditional mouse mutants is costly and time consuming due to extensive vector construction, ES cell manipulation, and breeding. The finding that RNAi can mediate potent gene knockdown in transgenic animals provides the opportunity to significantly reduce time and effort for the generation of genetically modified mouse models4-6.
RNAi application in transgenic mice
RNA interference (RNAi) has long been recognised as as a tool for inhibition of gene expression; it’s based on the introduction of double stranded RNA (dsRNA) molecules into cells, whereby one strand is complementary to the coding region of a target gene. Through pairing of the specific mRNA with the introduced RNA molecule, the mRNA is degraded by the RNAi machinery. Since long dsRNA provokes an interferon response in mammalian cells, the technology was initially restricted to organisms or cells showing no interferon response. The finding that short (<30 bp) interfering RNAs (siRNA) circumvent the interferon response extended the application to mammalian cells7. Sustained gene silencing has been achieved by the intracellular transcription of small inverted repeats separated by a spacer region. For the expression of these short hairpin RNAs (shRNAs), the RNA polymerase III dependent promoters U6 and H1 have been used in most cases. These promoters can be used to efficiently express shRNAs lacking non-functional bases at the 5’ and 3’ ends. The resulting shRNAs are processed in vivo into active ‘small interfering’ RNAs. Given that RNAi mediated gene knockdown have been known to reach efficiencies of 80% or more in mammalian cells, the technology promised great potential for gene function analysis in mice. Compared to the existing KO technology, RNAi requires just a single transgene to silence both alleles of the target gene. Furthermore, the required labour for generating shRNA transgenes is much lower compared to the construction of gene targeting vectors. Sustained, shRNA mediated RNAi in mice had been demonstrated in recent publications4-6, conditional, RNAi-mediated gene knockdown in mice has been introduced by using Cre/loxP for tissue specific control of shRNA expression8-10. The method of choice for precise gene function analyses in adult mice, however, is the temporal control of gene inactivation as it can prevent impaired embryonic development until the time of induction. Furthermore, it erradicates the investigation of the effects of gene inactivation after the onset of a chronic or acute disease. This aspect is of particular interest in the validation of genes for pharmaceutical drug development as it allows the inactivation of genes in the context of a fully developed disease background.
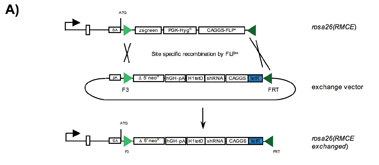
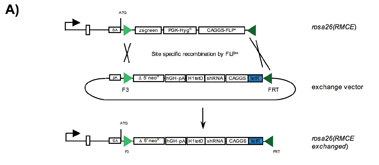
Figure 1: A) Single vector configuration for gene knockdown in vivo. RMCE through Flpe mediated recombination using the exchange vector generates the rosa26(RMCE exchanged) allele. The exchange vector carries the shRNA coding region under the control of the H1-tet promoter, the codon optimised itetR gene under the control of the CAGGS promoter, and a truncated neoR gene for positive selection of clones upon successful RMCE.
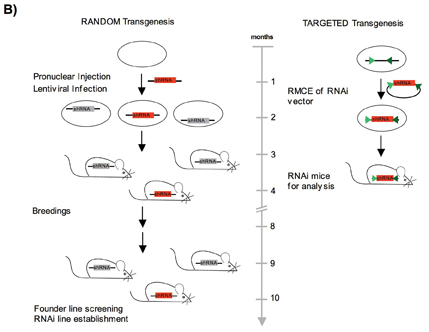
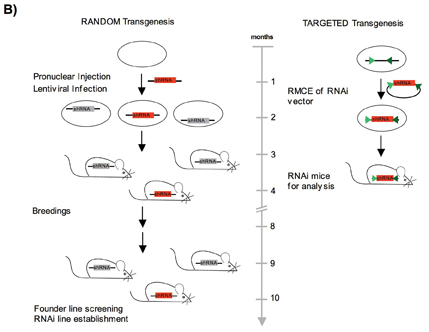
Figure 1: B) Generation of RNAi mice by a random or a targeted transgenesis approach. The red ‘shRNA’ box represents the RNAi expression vector, which can be inserted into the genome in a targeted or random way. By random insertion the transgene is inserted at various unknown positions within the genome and will be influenced by the surrounding chromatin. By the targeted approach the transgene is inserted always into the same genomic position and leads to a predictable shRNA expression for each construct.
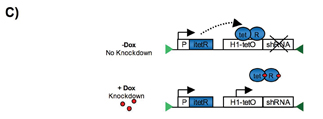
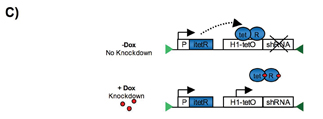
Figure 1: C) Schematic and simplified representation of the inducible system in a single vector cassette. The tetR (tet-repressor) inhibits the expression of the shRNA by binding to the tet-operator sequence. Dox (Doxycycline) leads to derepression of the repressor and switch on of the shRNA expression
Targeted transgenesis: the method of choice
Most of the published RNAi mouse models are based on random transgenesis via pronuclear injection; transfection of ES cells using plasmid based constructs or lentiviral infection4,5,8,10. However, the level and pattern of gene silencing in those animals was variable, presumably due to the inherent problem of position effect variegation. The expression varies significantly in most cases; depending on integration site, copy number and configuration of transgenes. In addition, founder mice occasionally fail to transmit the transgene. Furthermore, lentiviral infection usually results in multiple=”multiple” independent integrations into the genome that will segregate upon subsequent breeding of founder animals11,12. For these reasons, multiple=”multiple” founder lines need to be further mated and tested in order to identify a useful strain, which is particularly laborious and time-consuming13-17(Figure 1B). To address this complication, we tried to identify an autosomal locus supporting strong and predictable expression of shRNA transgenes upon insertion through site-specific recombination. The Rosa26 locus appeared most suitable for this purpose, as it had been described as being ubiquitously active throughout mouse development in every single cell of the body18,19. We therefore suspected that the open chromatin structure of Rosa26 might also promote the expression of shRNA constructs under the control of an RNA polymerase III dependent promoter. Our study revealed that a single copy of the shRNA transgene indeed mediates body wide gene silencing with high efficiency when inserted into Rosa26, ranging between 80-95% knock-down in most organs6. In the meantime we have reproduced this result by analysing the activity of more than 20 different shRNA constructs in vivo, demonstrating that the technology is robust and suitable for industrial application. RMCE technology platform To further accelerate the generation of shRNA transgenic animals, we adapted the method of ‘recombinase mediated cassette exchange’ (RMCE) to the Rosa26 locus (Figure 1A). Thus far, only a few examples of successful RMCE in ES cells had been described20,21. In these experiments, random integration of the exchange vector as well as incomplete recombination frequently produced unwanted transgene configurations. The efficiency of RMCE varied strongly, depending on the choice of recombination sites, the selection strategy, and the chromosomal target. We combined a couple of features to address these problems6 (Figure 1A): 1. We utilised Flp mediated RMCE using a wild type Flp target site (FRT) in combination with an inverted F3 site. The F3/F3 couple is recombined by FLP with the same efficiency as two wild type recombinase recognition sites (RRS) whereas recombination of a FRT/F3 pair is not catalyzed. This characteristic contrasts other pairs of wild type and mutant RRS, that were found to mediate a residual recombination activity. 2. We added a constitutive FLPe expression cassette on the targeting vector to provide sufficient recombinase activity until successful RMCE of the exchange vector. Incomplete recombination intermediates should therefore be avoided. 3. A 5’ truncated selection marker including a splice acceptor site but lacking a functional promoter was inserted into the exchange vector. Expression of the selection marker should therefore be mediated by the endogenous Rosa26 promoter following successful RMCE, but not through random integration of the exchange vector. We found that our refined RMCE strategy facilitates the insertion of shRNA transgenes into the Rosa26 locus with high efficiencies, consistently reaching a frequency of >90% correct clones6. The power of the RMCE technology has been validated by the insertion of more than 900 different vectors. Given the simple cloning strategy for the insertion of shRNA transgenes into the RMCE exchange vector and the limited screening efforts, our RMCE approach largely facilitates the generation of shRNA transgenic mice.
Further shortening the production timeline for RNAi based mouse models
The standard protocol for deriving mouse mutants currently requires the production of germline chimeras from targeted embryonic stem (ES) cells, followed by at least one breeding step to obtain a transgenic strain. The production of an shRNA transgenic mouse line is therefore a time intensive task; exceeding 7 months prior to the analysis of adult individuals. In order to shorten this timeline, we established the production of genetically engineered mouse models through introduction of diploid ES cells into tetraploid blastocysts. The latter provide an initial host environment for the differentiation of ES cells, but do not contribute to the embryo at later developmental stages. Although the methodology to produce such ‘ES cell-tetraploid’ (ES) mice from inbred ES cell lines had been described more than one decade ago22, its application was limited due to the extremely low frequency at which viable ES pups are recovered. In the meantime however, the technology was significantly improved through the discovery of Artemis’ scientific advisor, Rudolf Jaenisch (Whitehead Institute, Cambridge, MA); ES cell lines derived from hybrid mouse strains support the development of viable ES mice at 50-fold higher rate as compared to inbred ES cells23. Thus, the ‘ES mouse technology’ offers the opportunity to efficiently produce targeted mouse mutants directly from hybrid ES cell clones within the time of a single mouse generation and without the requirement of further breeding. By combining the tetraploid blastocyst complementation approach with RMCE for targeted insertion of shRNA transgenes into the ES cell genome, we now reach a timeline of less than 4 months for the generation of adult shRNA transgenic mouse models6,24. Moreover, the strategy should be applicable to other genetic model organisms such as rats, in which homologous recombination is not yet established for routine application.
Inducible shRNA expression
The method of choice for precise gene function analyses in adult mice is the temporal control of gene silencing as it can prevent impaired embryonic development until the time of induction. In particular, inducible gene silencing in all organs would be a useful tool if the major site of gene action is not precisely defined. Optimally, such a system should provide a reversible gene switch upon every single cell of the body.
In cultured cell lines, inducible expression of antisense or short hairpin RNAs have been achieved by using engineered, RNA polymerase III-dependent promoters containing operator sequences (tetO) of the E. coli tetracycline resistance operon25-28. Binding of the tetracycline resistance operon repressor (tetR) to tetO blocks transcription, whereas de-repression is achievable by adding the inductor doxycycline (dox), causing release of TetR (Figure 1C).
We have recently shown that a codon optimised tet repressor, not the wt coding region as employed in previous attempts, facilitates tight control of the H1 promoter when integrated at the Rosa26 locus24. We found efficient, doxycycline-inducible gene silencing within 10 days of doxycycline treatment, reaching 80-90% repression in most tissues. As expected, the system appeared to be reversible upon withdrawal of the inductor. Combined with RMCE and tetraploid blastocysts complementation, our system facilitates a rapid performance of in vivo gene function studies, providing a generally applicable tool for reverse mouse genetics research. Moreover, it permits the investigation of the effect of gene knockdown after the onset of a chronic or acute disease. This aspect is of particular interest for pharmaceutical drug target validation, as inducible gene silencing in mouse models of human disease can provide an ideal surrogate for the treatment of patients with antagonistic drugs.
References
- Gu H, Marth JD, Orban PC, Mossmann H, Rajewsky K. (1994) Deletion of a DNA polymerase beta gene segment in T cells using cell type-specific gene targeting. Science, 265:103-6.
- Glaser, S., Anastassiadis, K. and Stewart, A.F. (2005) Current issues in mouse genome engineering. Nat Genet. 37,1187-1193.
- Kühn, R. and Schwenk, F. (2003) Conditional knockout mice. Methods Mol Biol., 209, 159-185.
- Carmell, M.A., Zhang, L., Conklin, D.S., Hannon, G.J. and Rosenquist, T.A. (2003) Germline transmission of RNAi in mice. Nat Struct Biol., 10, 91-92.
- Rubinson, D.A., Dillon, C.P., Kwiatkowski, A.V., Sievers, C., Yang, L. Kopinja, J., Rooney, D. L., Ihrig, M. M., McManus, M. T. and Gertler F. B. (2003) A lentivirus-based system to functionally silence genes in primary mammalian cells, stem cells and transgenic mice by RNA interference. Nat Genet., 33, 401-406.
- Seibler, J., Kuter-Luks, B., Kern, H., Streu, S., Plum, L., Mauer, J., Kuhn, R., Bruning, J. C. and Schwenk F. (2005) Single copy shRNA configuration for ubiquitous gene knockdown in mice. Nucleic Acids Res., 33, e67.
- Elbashir SM, Harborth J, Lendeckel W, Yalcin A, Weber K, Tuschl T. Nature. 2001 May 24; 411(6836):494-8.
- Ventura, A., Meissner, A., Dillon, C.P., McManus, M., Sharp, P.A., Van Parijs, L., Jaenisch, R. and Jacks, T. (2004) Cre-lox-regulated conditional RNA interference from transgenes. Proc. Natl. Acad. Sci. USA, 101, 10380-10385.
- Oberdoerffer, P., Kanellopoulou, C., Heissmeyer, V., Paeper, C., Borowski, C., Aifantis, I., Rao, A. and Rajewsky, K. (2005) Efficiency of RNA interference in the mouse hematopoietic system varies between cell-types and developmental stages. Mol Cell Biol., 25, 3896-905.
- Coumoul, X., Shukla, V., Li, C., Wang, R.H. and Deng, C.X. (2005) Conditional knockdown of Fgfr2 in mice using Cre-LoxP induced RNA interference. Nucleic Acids Res., 33, e102.
- van den Brandt J, Wang D, Kwon SH, Heinkelein M, Reichardt HM. Lentivirally generated eGFP-transgenic rats allow efficient cell tracking in vivo. Genesis. 2004 Jun;39(2):94-9.
- Chen Z, Stockton J, Mathis D, Benoist C. Proc Natl Acad Sci U S A. 2006 Oct 31;103(44):16400-5. Epub 2006 Oct 23.
- Bradley & Liu (1996) Target practice in transgenics. Nat Genetics, 14: 121-123.
- Jasin et al., Targeted transgenesisPNAS 1996, 93: 8804-08.
- Dobie et al. (1997) Variegated gene expression in mice. Trends Genet, 13(4): 127-30.
- Garrick et al., (1998) Repeat-induced gene silencing in mammals. Nat Genet 1998 Jan;18(1):56-9.
- Al-Shawi et al., Mol Cell Biol 1990,10(3):1192-8.
- Soriano, P. (1999) Generalized lacZ expression with the ROSA26 Cre reporter strain. Nat Genet. 21, 70-71.
- Seibler, J., Zevnik, B., Kuter-Luks, B., Andreas, S., Kern, H., Hennek, T., Rode, A., Heimann, C., Faust, N., Kauselmann, G. et al. Nucleic Acids Res. 31 (2003) e12.
- Seibler J., Schubeler D., Fiering S., Groudine M. and Bode J. (1998) DNA cassette exchange in ES cells mediated by Flp recombinase: an efficient strategy for repeated modification of tagged loci by marker-free constructs. Biochemistry 37, 6229-6234.
- Feng Y.Q., Seibler J., Alami R., Eisen A., Westerman K.A., Leboulch P., Fiering S. and Bouhassira E.E. (1999) Site-specific chromosomal integration in mammalian cells: highly efficient CRE recombinase-mediated cassette exchange. J Mol Biol. 292, 779-785.
- Nagy, A., Rossant, J., Nagy, R., Abramov-Newerly, W. & Roder, J. C. (1993) Proc. Natl. Acad. Sci. USA 90, 8424–8428.
- Eggan, K., Akutsu, H., Loring, J., Jackson-Grusby, L., Klemm, M., Rideout, W.M. 3rd, Yanagimachi R. and Jaenisch, R. (2001) Hybrid vigor, fetal overgrowth, and viability of mice derived by nuclear cloning and tetraploid embryo complementation. Proc. Natl. Acad. Sci. USA, 98, 6209-6214.
- Seibler J., Kleinridders A., Küter-Luks B., Niehaves S., Brüning J.C. & Schwenk F. (2007) Generation of a reversible type 2 Diabetes mellitus mouse model by transgenic, doxycycline regulated shRNA expression. Nucleic Acids Res. 2007;35(7):e54. Epub 2007 Mar 21.
- Ohkawa, J. and Taira, K. (2000) Control of the functional activity of an antisense RNA by a tetracycline-responsive derivative of the human U6 snRNA promoter. Hum Gene Ther., 11, 577-85.
- van de Wetering, M., Oving, I., Muncan, V., Pon Fong, M.T., Brantjes, H., van Leenen, D., Holstege, F. C., Brummelkamp, T. R., Agami, R. and Clevers, H. (2003) Specific inhibition of gene expression using a stably integrated, inducible small-interfering-RNA vector. EMBO Rep., 4, 609-615.
- Matsukura, S., Jones, P.A. and Takai, D. (2003) Establishment of conditional vectors for hairpin siRNA knockdowns. Nucleic Acids Res., 31, e77.
- Czauderna, F., Santel, A., Hinz, M., Fechtner, M., Durieux, B., Fisch, G., Leenders, F., Arnold, W., Giese, K., Klippel, A. and Kaufmann J. (2003) Inducible shRNA expression for application in a prostate cancer mouse model. Nucleic Acids Res., 31, e127.
Jost Seibler
Head of Technology Development, Artemis Pharmaceuticals
Jost Seibler developed recombinase based technologies for genetic engineering of the ES cell genome, including a system for the efficient exchange of gene expression cassettes at the National Research Center for Biotechnology in Braunschweig (GBF). He received his Ph.D in 1999 from the TU-Braunschweig. At ARTEMIS Pharmaceuticals, Cologne, Jost Seibler leads the group dedicated towards the development of techniques for in vivo RNAi gene knockdown. He developed novel and efficient systems for inducible and reversible shRNA expression in mice. At ARTEMIS he has contributed significantly towards development of proprietary knock out systems and other key technologies.
Frieder Schwenk
Principal Scientist, University of Applied Science, Department of Applied Natural Sciences, Gelsenkirchen, Germany
Frieder Schwenk, Ph.D., Principal Scientist is a highly renowned expert in the field of mouse genetics and remains associated with ARTEMIS in a part-time capacity. Frieder Schwenk was the head of the technology department at ARTEMIS Pharmaceuticals till October 2003. He joined ARTEMIS in 1998 from the laboratory of Dr. Klaus Rajewsky, where he made a major contribution to the pioneering work on the conditional gene targeting strategy. Dr. Schwenk received his Ph.D in Genetics in 1997 from the University of Cologne on the development of the first system for temporally and spatially regulated gene targeting in mice. He holds a professorship in Molecular Biology and Physiology at the University of Applied Science in Gelsenkirchen, Germany.