The expanding world of small RNA: from germ cells to cancer
Posted: 21 September 2007 |
Over the last ten years a small RNA revolution has swept biology. In 1998 RNA interference (RNAi) was discovered as an experimental tool by Andy Fire and Craig Mello, a finding that was awarded with the 2006 Nobel Prize for Physiology or Medicine. Although the biology of RNAi is still not understood, it has become a powerful experimental tool and is currently being developed for human gene therapy. During a similar time-frame and linked in some aspects to RNAi, microRNAs (miRNAs) were discovered as a new class of regulatory RNAs in animals, plants and viruses.
Over the last ten years a small RNA revolution has swept biology. In 1998 RNA interference (RNAi) was discovered as an experimental tool by Andy Fire and Craig Mello, a finding that was awarded with the 2006 Nobel Prize for Physiology or Medicine. Although the biology of RNAi is still not understood, it has become a powerful experimental tool and is currently being developed for human gene therapy. During a similar time-frame and linked in some aspects to RNAi, microRNAs (miRNAs) were discovered as a new class of regulatory RNAs in animals, plants and viruses.
Over the last ten years a small RNA revolution has swept biology. In 1998 RNA interference (RNAi) was discovered as an experimental tool by Andy Fire and Craig Mello1, a finding that was awarded with the 2006 Nobel Prize for Physiology or Medicine. Although the biology of RNAi is still not understood, it has become a powerful experimental tool and is currently being developed for human gene therapy2. During a similar time-frame and linked in some aspects to RNAi, microRNAs (miRNAs) were discovered as a new class of regulatory RNAs in animals, plants and viruses3.
MicroRNAs are transcribed from endogenous genes as long, primary RNA transcripts and are processed to their mature form: a single-stranded RNA with a length of approximately 22 nucleotides, indistinguishable from a small-interfering RNA (siRNA), the mediator of RNAi (Figure 1). In animals these long RNA precursors (pri-miRNAs)4 are processed in the nucleus by the RNase III enzyme Drosha and Pasha/DGCR8 to form the approximately 70-base pre-miRNAs5. Pre-miRNAs are exported from the nucleus by Exportin-56, processed by the RNase III enzyme Dicer, and incorporated into an Argonaute-containing silencing complex (RISC)7. MicroRNAs are thought to regulate gene expression post-transcriptionally by forming Watson-Crick base pairs with target miRNAs. Their mechanism of action is still under debate, but likely includes inhibition of translation and mRNA degradation8. In animals, most miRNAs are thought to form imperfect base-pairs with their target mRNA(s) and these interaction sites are enriched in 3’ un-translated regions (3’UTRs)3. As a consequence, miRNA target identification using computational approaches is non-trivial9. The public database for miRNAs, miRBase release 9.2, currently lists 533 human microRNAs10 and estimates for the total number of human microRNAs range from over one thousand11 to tens of thousands12. Although miRNAs have only been studied intensely for the last five years, important functions for miRNAs in animal development and, potentially, human disease, have already emerged13.
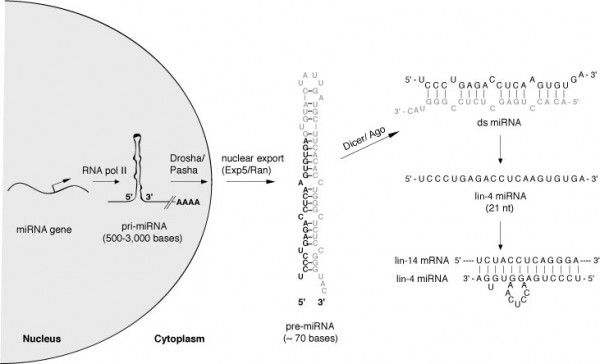
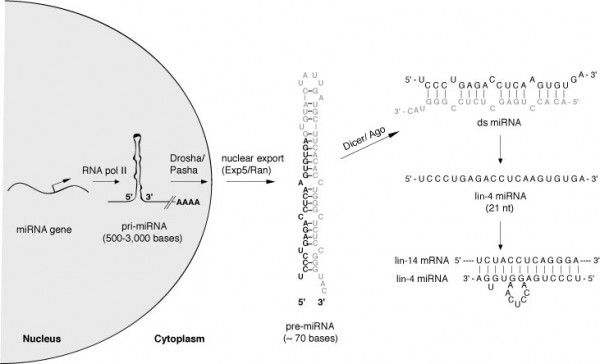
Figure 1: MicroRNA biogenesis and mechanism of action. A current model of microRNA biogenesis and mechanism of action. This model is a generic model of the biogenesis of microRNAs in animals. The lin-4 miRNA and the lin-14 mRNA are shown as examples.
This review focuses on the potential roles for miRNAs and piRNAs in human cancer and their potential for diagnostics, prognostics and therapy.
MicroRNA misexpression in cancer
The first evidence for a direct link between miRNAs and human cancer came from the observation that two microRNA genes, mir-15 and mir-16 are located in a 30 kilobase region on chromosome 13 that had been found deleted in chronic lymphocytic leukaemia (CLL) cases, and that miR-15 and miR-16 expression is often reduced in CLL14. A second study found that miR-143 and miR-145 expression levels were reduced in adenomatous and cancer stages of colorectal neoplasia15. Both studies were focused on a small number of miRNAs and based on miRNA cloning and northern blotting approaches. Subsequent development of a number of miRNA microarray technologies coincided with an increased number of miRNA expression studies in human cancer16-17. Crucially, quantitative reverse transcriptase polymerase chain reaction (qRT-PCR) for mature miRNAs have also become available for the analysis of small tissue samples and microarray miRNA validation18. A current map of all miRNA loci implicated in at least two different human cancers through expression profiling lists 56 such loci. However, the number of miRNAs implicated in human cancer by expression profiling is likely to continue to increase substantially for two reasons: firstly, the recent availability of commercial miRNA profiling platforms will widen access to these tools and secondly, the number of known human miRNAs is still increasing, rendering even the most recently published expression studies incomplete. Some general themes from the expression profiles published to date are emerging. For example, a study of 334 primary human tumours and tissues interrogating the expression of 217 miRNAs, revealed that miRNA expression profiles contain lineage-specific information, may classify even poorly differentiated tumours, and that many miRNAs are down-regulated in primary tumours as compared to normal tissues17. The notion that a common set of miRNAs may be deregulated in many tumour types is also supported by the finding that a large number of miRNAs have been implicated in at least two distinct tumour types. It is tempting to speculate that these miRNAs share anti-tumourgenic properties. It is unknown how miRNA expression in tumours may be de-regulated. The development of in situ approaches to directly visualise microRNAs in human tumour cells using oligonucleotide probes, which have been successful in plants, flies and fish19, may help address the question if miRNA expression is altered stochastically or co-ordinately, is cell-specific or tumour-wide.
MicroRNAs as tumour suppressors and oncogenes?
Two decades ago cell-based transformation assays identified the first human oncogenes and tumour suppressors20. More recently, a cell-based screen identified miR-372 and miR-373 out of 197 miRNAs as putative oncogenes in a RAS co-operation assay21. This study implicated miR-372 and miR-373 in the p53 pathway with the tumour suppressor LATS2 as a possible direct target and suggested a potential role for these miRNAs in testicular germ cell tumours. A number of other studies have taken specific miRNAs identified in expression profiling experiments forward to carry out functional analyses. For example, miR-21 was found to be over-expressed in malignant cholangiocytes and miR-21 expression was found to down-regulate the tumour suppressor PTEN in these cells22. In a separate study the microRNAs miR-20 and miR-106a were found to be able to regulate the tumour suppressors TGFBR2 and RB, respectively23. All of the above, and work on the miR-17 to -92 cluster of miRNAs that will be discussed in the next section, suggest that a number of miRNAs may have oncogenic potential. However, several miRNAs have been proposed to act as tumour suppressors. The first of these included members of the let-7 family, which were reported to regulate the expression of the RAS oncogene in the nematode Caenorhabditis elegans and in human cells24. The same study found let-7 to be down-regulated in lung tumours and its expression anti-correlated with that of RAS. This observation was particularly intriguing given the functional studies of the let-7 miRNA in C. elegans. The let-7 family was subsequently found to be de-regulated in large number of tumour types and the oncogenes HMGA2 and FOS have been added to its list of putative targets25. Other oncogenes that may be targeted directly by miRNAs include; AIB1, BCL2, BCL6, E2F3, KIT and TCL126-29. Interestingly, miR-21 was shown to be able to regulate the oncogene PTEN in cholangiocytes22 and the tumour suppressor BCL2 in the breast cancer cell line MCF-727, suggesting that miR-21 may act as a tumour suppressor or oncogene depending on cellular context.
The mir-19-92 “oncomir” cluster
If the let-7 miRNA is the best-studied candidate tumour suppressor miRNA, the mir-17-92 miRNA polycistron is the best-studied miRNA with oncogenic potential and was named “OncomiR-1”. The mir-17-92 cluster (c13orf25) is part of a region on chromosome 13 amplified in malignant lymphoma including B-cell lymphoma30. Using a well-established mouse model of B-cell lymphoma in which haematopoietic stem cells over-expressing the oncogenic transcription factor Myc, are used to generate mosaic animals it was demonstrated that mice mosaic for cells over- expressing Myc and the mir-17-92 cluster, developed tumours earlier than mice with cells over-expressing Myc alone31. Furthermore Myc and mir-17-92 cluster induced tumours were more aggressive with higher mitotic indices than Myc-only induced tumours31. An independent study demonstrated that Myc directly binds to and regulates the transcription of the mir-17-92 cluster in cell culture and that the miRNAs of this cluster regulate the expression of E2f132. These two studies suggested a complex network of regulation whereby MYC, which is a positive regulator of E2F1 transcription, dampens the E2F1 response through the miRNAs of the mir-17-92 cluster. However, the picture is likely more complex involving several E2F proteins (E2F1, E2F2, E2F3), feedback loops from E2F to MYC and from E2F to the mir-17-92 cluster and affects on angiogenesis and tumour metasteses by targeting TSP1, CTGF and TGFBR223,29,32,33.
MicroRNA germline mutations
Given the data implicating miRNAs in cancer reviewed here, one might expect germline mutations in miRNAs to be involved in cancer pre-disposition. Indeed, single nucleotide polymorphisms (SNPs) in the primary transcripts of miR-15a and mIR-16-1 may be linked to CLL34. But the net should be cast much wider to include miRNA targets. Our current understanding of miRNA interaction with target mRNAs suggests that a single 3’UTR mutation might make or break the miRNA interaction9. The importance of mutations in miRNA binding site for inheritable traits has already been demonstrated in the case of Texel sheep, where a quantitative trait locus maps to a mutation in the 3’UTR of the muscle-specific gene GDF8, which renders it a target for the miRNAs miR-1 and miR-20635. MiRNA target site mutations have also been shown to be of potential importance for human disease as a mutation in a putative miR-189 binding-site in human SLITRK1 may be linked to Tourette’s syndrome36. Interestingly, SNPs in the binding sites for the miRNAs miR-146, miR-221 and miR-222 in the oncogene KIT may be linked to papillary thyroid carcinoma28. However, a recent study identified a number of SNPs in primary miRNA sequences, one in the pre-miRNA for miR-26-a1, and none in mature miRNAs in a panel of 91 human cancer cell lines37. None of the miRNA associated SNPs resulted in changes in miRNA expression or processing37.
Novel small RNAs: piRNAs regulate germline development
MicroRNAs are no longer the only small endogenous RNA species (Figure 2). Repetitive elements of animal, plant and fungal genomes are the source of repeat-associated small interfering RNAs (rasiRNAs, or endo-siRNAs). These RNAs are likely derived from double-stranded precursors and while they play important roles in the silencing of repeat elements in fungal, plant and invertebrate genomes, their role in mammals is not well understood. In addition, a novel class of small RNAs was discovered last year in the germ-line of many animals; including flies, fish and mammals38. These PIWI-associated RNAs (piRNAs) appear to have a biogenesis pathway distinct from miRNAs and endogenous siRNAs, which has yet to be elucidated. Similarly, the mechanism by which piRNAs act is unknown. However, the reason that this new RNA species has generated a lot of interest is that it is required for the maintenance of germ-line stem cells in flies, fish and mice38. Many piRNAs map to transposable elements in the genome and at least part of their role is to silence expression and mobility of these elements in the germline38. Therefore, piRNAs directly contribute to fertility and germ-line genome stability. These observations suggest that piRNAs may have important roles in cancer, in particular germline cancers.
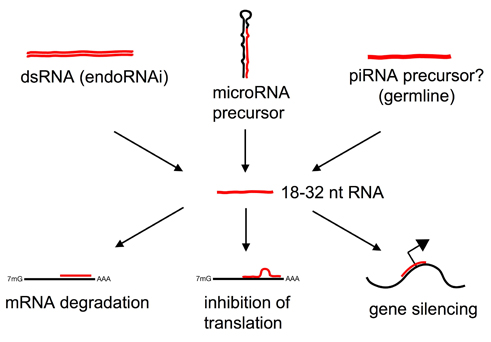
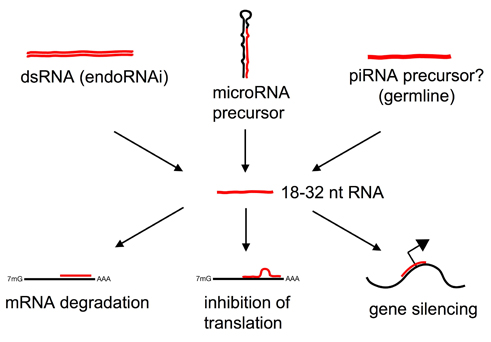
Figure 2: Short RNAs as key regulators of gene expression. Endogenous short regulatory RNAs may be derived from double-stranded precursors transcribed from repetitive elements (endo-siRNAs, rasiRNAs), RNAPolII transcripts (microRNAs) or in the germline from unknown precursors (piRNAs). Short regulatory RNAs may regulate RNA degradation, mRNA translation or possibly gene transcription.
Diagnostics
The body of expression data for miRNAs in cancer available to date suggests that miRNAs may have diagnostic potential. However, while initial expression studies focused on comparing normal tissues to tumours, to gauge diagnostic potential it will be more important to correlate miRNA expression with tumour subtypes or clinical parameters. A number of studies show promise in this regard. For example, a qRT-PCR based study identified subsets of miRNAs that distinguish ErbB2-positive from ErbB2-negative and ER-positive from ER-negative breast cancers from biopsies39. If miRNA expression data can be used to build discriminators with clinical value, miRNAs have clear advantages over mRNAs: they are long-lived in vivo40 and very stable in vitro.
Therapy
Given the emerging evidence of miRNAs with oncogenic or tumour suppressor activities, it is important to seek routes to interfere with miRNAs and to develop these as novel cancer therapies. In the case of the oncogenic mir-17-92 cluster it might therefore be of interest to specifically knockdown the expression of all miRNAs derived from this cluster. In mammalian cell culture 2-O-Methyl oligoribonucleotides (2-O-Me-RNA) have been used to specifically down-regulate miRNAs41. Cell-permeable forms of these 2-O-Me-RNAs, called “antagomirs” were successful in down-regulating several mouse miRNAs in a number of mouse tissues following intravenous injection in vivo42. It remains an open question whether these or similar strategies will allow us to deliver anti-miRNA cancer therapies. One complication that may make the task more difficult is the potential redundancy of miRNAs: would targeting mir-17 be sufficient or would all miRNAs of the mir-17-92 cluster have to be targeted? In contrast, for miRNAs that act as tumour suppressors, it may be of interest to develop in vivo expression systems. As miRNAs are chemically identical to siRNAs ongoing efforts to deliver siRNAs as RNAi-based anti-cancer therapies, if successful, should provide suitable vehicles for the delivery of miRNAs2. In fact, some methods of siRNA delivery make use of the miRNA biogenesis pathway to increase efficiency2. Even if the delivery problem may be solved, one major obstacle to the efficacy of siRNA or miRNA based therapies may be the potential of off-target effects43. Finally, inhibiting miRNA expression globally by interfering with their biogenesis may have therapeutic potential just as inhibiting global chromatin-modifying enzymes such as histone deacetylases (HDACs) is proving effective in some types of cancer44.
Conclusion
The last six years have demonstrated the importance of miRNA biology. The evidence for roles of miRNAs in human disease, in particular cancer, is overwhelming. Last year’s discovery of a new RNA species, the germ-line restricted piRNAs, suggest that miRNAs were just the tip of the iceberg. Although initially in the shadow of RNAi, endogenous small RNAs have become the brightest star of the small RNA revolution.
References
- A. Fire, S. Xu, M. K. Montgomery et al., Nature 391 (6669), 806 (1998).
- D. Bumcrot, M. Manoharan, V. Koteliansky et al., Nature chemical biology 2 (12), 711 (2006).
- D. P. Bartel, Cell 116 (2), 281 (2004).
- V. N. Kim, Nat Rev Mol Cell Biol 6 (5), 376 (2005).
- Y. Lee, C. Ahn, J. Han et al., Nature 425 (6956), 415 (2003); M. Landthaler, A. Yalcin, and T. Tuschl, Curr Biol 14 (23), 2162 (2004); J. Han, Y. Lee, K. H. Yeom et al., Genes Dev 18 (24), 3016 (2004); R. I. Gregory, K. P. Yan, G. Amuthan et al., Nature 432 (7014), 235 (2004); A. M. Denli, B. B. Tops, R. H. Plasterk et al., Nature 432 (7014), 231 (2004).
- E. Lund, S. Guttinger, A. Calado et al., Science 303 (5654), 95 (2004).
- T. Du and P. D. Zamore, Development 132 (21), 4645 (2005).
- R. J. Jackson and N. Standart, Sci STKE 2007 (367), re1 (2007).
- B. John, C. Sander, and D. S. Marks, Methods Mol Biol 342, 101 (2006).
- S. Griffiths-Jones, Nucleic Acids Res 32 (Database issue), D109 (2004); S. Griffiths-Jones, R. J. Grocock, S. van Dongen et al., Nucleic Acids Res 34 (Database issue), D140 (2006).
- E. Berezikov, V. Guryev, J. van de Belt et al., Cell 120 (1), 21 (2005).
- K. C. Miranda, T. Huynh, Y. Tay et al., Cell 126 (6), 1203 (2006).
- I. Alvarez-Garcia and E. A. Miska, Development 132 (21), 4653 (2005).
- G. A. Calin, C. D. Dumitru, M. Shimizu et al., Proc Natl Acad Sci U S A 99 (24), 15524 (2002).
- M. Z. Michael, O’ Connor SM, N. G. van Holst Pellekaan et al., Mol Cancer Res 1 (12), 882 (2003).
- A. M. Krichevsky, K. S. King, C. P. Donahue et al., Rna 9 (10), 1274 (2003); G. A. Calin, C. G. Liu, C. Sevignani et al., Proc Natl Acad Sci U S A 101 (32), 11755 (2004); E. A. Miska, E. Alvarez-Saavedra, M. Townsend et al., Genome Biol 5 (9), R68 (2004); J. M. Thomson, J. Parker, C. M. Perou et al., Nat Methods 1 (1), 47 (2004); C. G. Liu, G. A. Calin, B. Meloon et al., Proc Natl Acad Sci U S A 101 (26), 9740 (2004).
- J. Lu, G. Getz, E. A. Miska et al., Nature 435 (7043), 834 (2005).
- R. Shi and V. L. Chiang, Biotechniques 39 (4), 519 (2005); F. Tang, P. Hajkova, S. C. Barton et al., Nucleic Acids Res 34 (2), e9 (2006).
- C. A. Kidner and R. A. Martienssen, Nature 428 (6978), 81 (2004); N. S. Sokol and V. Ambros, Genes Dev 19 (19), 2343 (2005); E. Wienholds, W. P. Kloosterman, E. Miska et al., Science 309 (5732), 310 (2005).
- S.H. Friend, T.P. Dryia, and R. A. Weinberg, N Engl J Med 318 (10), 618 (1988).
- P. M. Voorhoeve, C. le Sage, M. Schrier et al., Cell 124 (6), 1169 (2006).
- F. Meng, R. Henson, M. Lang et al., Gastroenterology 130 (7), 2113 (2006).
- S. Volinia, G. A. Calin, C. G. Liu et al., Proc Natl Acad Sci U S A 103 (7), 2257 (2006).
- S. M. Johnson, H. Grosshans, J. Shingara et al., Cell 120 (5), 635 (2005).
- H. J. Lee, M. Palkovits, and W. S. Young, 3rd, Proc Natl Acad Sci U S A 103 (42), 15669 (2006); T. Wang, X. Zhang, L. Obijuru et al., Genes Chromosomes Cancer 46 (4), 336 (2007); C. Hebert, K. Norris, M. A. Scheper et al., Mol Cancer 6, 5 (2007).
- C. Welch, Y. Chen, and R. L. Stallings, Oncogene (2007); Y. Pekarsky, U. Santanam, A. Cimmino et al., Cancer Res 66 (24), 11590 (2006); Y. Saito, G. Liang, G. Egger et al., Cancer Cell 9 (6), 435 (2006); A. Cimmino, G. A. Calin, M. Fabbri et al., Proc Natl Acad Sci U S A 102 (39), 13944 (2005); N. Felli, L. Fontana, E. Pelosi et al., Proc Natl Acad Sci U S A 102 (50), 18081 (2005); L. Poliseno, A. Tuccoli, L. Mariani et al., Blood 108 (9), 3068 (2006).
- M. L. Si, S. Zhu, H. Wu et al., Oncogene (2006).
- H. He, K. Jazdzewski, W. Li et al., Proc Natl Acad Sci U S A 102 (52), 19075 (2005).
- A. Hossain, M. T. Kuo, and G. F. Saunders, Mol Cell Biol 26 (21), 8191 (2006).
- A. Ota, H. Tagawa, S. Karnan et al., Cancer Res 64 (9), 3087 (2004).
- L. He, J. M. Thomson, M. T. Hemann et al., Nature 435 (7043), 828 (2005).
- K. A. O’Donnell, E. A. Wentzel, K. I. Zeller et al., Nature 435 (7043), 839 (2005).
- C. L. Wang, B. B. Wang, G. Bartha et al., Proc Natl Acad Sci U S A 103 (49), 18680 (2006); K. Woods, J. M. Thomson, and S. M. Hammond, J Biol Chem 282 (4), 2130 (2007); L. Venturini, K. Battmer, M. Castoldi et al., Blood (2007); M. Dews, A. Homayouni, D. Yu et al., Nat Genet 38 (9), 1060 (2006); Y. Sylvestre, V. De Guire, E. Querido et al., J Biol Chem 282 (4), 2135 (2007); Y. Hayashita, H. Osada, Y. Tatematsu et al., Cancer Res 65 (21), 9628 (2005).
- G. A. Calin, M. Ferracin, A. Cimmino et al., N Engl J Med 353 (17), 1793 (2005).
- A. Clop, F. Marcq, H. Takeda et al., Nat Genet 38 (7), 813 (2006).
- J. F. Abelson, K. Y. Kwan, B. J. O’Roak et al., Science 310 (5746), 317 (2005).
- S. Diederichs and D. A. Haber, Cancer Res 66 (12), 6097 (2006).
- A. G. Seto, R. E. Kingston, and N. C. Lau, Mol Cell 26 (5), 603 (2007).
- M. D. Mattie, C. C. Benz, J. Bowers et al., Mol Cancer 5, 24 (2006).
- L. P. Lim, N. C. Lau, P. Garrett-Engele et al., Nature 433 (7027), 769 (2005).
- G. Hutvagner, M. J. Simard, C. C. Mello et al., PLoS Biol 2 (4), e98 (2004).
- J. Krutzfeldt, N. Rajewsky, R. Braich et al., Nature 438 (7068), 685 (2005).
- A. L. Jackson, S. R. Bartz, J. Schelter et al., Nat Biotechnol 21 (6), 635 (2003); A. L. Jackson, J. Burchard, J. Schelter et al., Rna 12 (7), 1179 (2006).
- N. Carey and N. B. La Thangue, Current opinion in pharmacology 6 (4), 369 (2006).
Eric A. Miska
The Wellcome Trust/Cancer Research UK Gurdon Institute and Department of Biochemistry, University of Cambridge, Cambridge, UK
Eric Miska is a Cancer Research UK Senior Fellow working at the Wellcome Trust/Cancer Research UK Gurdon Institute at the University of Cambridge, UK. He received a BA from Trinity College, Dublin, a PhD from the University of Cambridge and was a postdoctoral fellow at the Massachusetts Institute of Technology, Cambridge, MA, USA.