Applications of MRI to controlled drug delivery devices
Posted: 22 October 2012 |
Magnetic resonance imaging (MRI) is a technique that is traditionally used as a diagnostic clinical imaging tool. However, there are now an increasing number of non-medial applications where MRI has seen unrivalled success. One of those areas is in its application to pharmaceutical research. The aim of this article is to briefly outline the quantitative nature of MRI and how it has been used recently to quantify dissolution phenomena in controlled drug delivery tablets under pharmacopeial conditions.
In modern pharmacotherapy, the effectiveness of a therapeutic treatment does not rely solely on the efficacy of the active pharmaceutical ingredient (API), but is also dependent upon a suitable dosage form or drug delivery device being available. In many ways, it is the drug delivery device itself that ensures the active drug is available at the site of action for the correct time and duration with appropriate drug concentration1. Drug delivery systems can be broadly divided into two categories according to their mechanisms of drug release: immediate release and modified release. Immediate release dosage forms, such as traditional painkillers, are relatively simple systems and are designed to release APIs instantaneously, i.e. when a fast therapeutic action is required. Modified release dosage forms, which are generally more suited to disease treatment, are generally designed to provide targeted / tailored drug release characteristics and a detailed knowledge of the spatio-temporal behaviour of the API is extremely important.
Magnetic resonance imaging (MRI) is a technique that is traditionally used as a diagnostic clinical imaging tool. However, there are now an increasing number of non-medial applications where MRI has seen unrivalled success. One of those areas is in its application to pharmaceutical research. The aim of this article is to briefly outline the quantitative nature of MRI and how it has been used recently to quantify dissolution phenomena in controlled drug delivery tablets under pharmacopeial conditions.In modern pharmacotherapy, the effectiveness of a therapeutic treatment does not rely solely on the efficacy of the active pharmaceutical ingredient (API), but is also dependent upon a suitable dosage form or drug delivery device being available. In many ways, it is the drug delivery device itself that ensures the active drug is available at the site of action for the correct time and duration with appropriate drug concentration1. Drug delivery systems can be broadly divided into two categories according to their mechanisms of drug release: immediate release and modified release. Immediate release dosage forms, such as traditional painkillers, are relatively simple systems and are designed to release APIs instantaneously, i.e. when a fast therapeutic action is required. Modified release dosage forms, which are generally more suited to disease treatment, are generally designed to provide targeted / tailored drug release characteristics and a detailed knowledge of the spatio-temporal behaviour of the API is extremely important.
Magnetic resonance imaging (MRI) is a technique that is traditionally used as a diagnostic clinical imaging tool. However, there are now an increasing number of non-medial applications where MRI has seen unrivalled success. One of those areas is in its application to pharmaceutical research. The aim of this article is to briefly outline the quantitative nature of MRI and how it has been used recently to quantify dissolution phenomena in controlled drug delivery tablets under pharmacopeial conditions.
In modern pharmacotherapy, the effectiveness of a therapeutic treatment does not rely solely on the efficacy of the active pharmaceutical ingredient (API), but is also dependent upon a suitable dosage form or drug delivery device being available. In many ways, it is the drug delivery device itself that ensures the active drug is available at the site of action for the correct time and duration with appropriate drug concentration1. Drug delivery systems can be broadly divided into two categories according to their mechanisms of drug release: immediate release and modified release. Immediate release dosage forms, such as traditional painkillers, are relatively simple systems and are designed to release APIs instantaneously, i.e. when a fast therapeutic action is required. Modified release dosage forms, which are generally more suited to disease treatment, are generally designed to provide targeted / tailored drug release characteristics and a detailed knowledge of the spatio-temporal behaviour of the API is extremely important. Modified release dosage forms can be sub-divided into two main categories. Delayed release dosage forms where the system is designed to release the active ingredient at a specific time after ingestion, but not immediately after administration, and extended dosage forms where the system is formulated to release the active ingredient over prolonged time periods.
A controlled release delivery system offers a sustained release profile but is designed to obtain a predictably constant plasma concentration. Unlike sustained release systems, which are restricted to oral dosage forms, controlled release systems use other administration routes, e.g. transdermal. Of central importance during the research and development of modified / controlled release drug delivery systems is the ability to better understand the results obtained from traditional pharmaceutical dissolution testing. Rigorous dissolution testing is a vital component of the whole drug development process, but it is fair to say that is still a rather empirical and inefficient method. Conventional dissolution testing provides limited insights into the local mass transport of a drug during its passage from the solid drug delivery device into the surrounding dissolution medium. Whilst dissolution testing is extremely valuable, it would be much more advantageous to be able to design and test controlled release formulations using a quality by design approach (QbD). In this way, the number of formulations requiring dissolution testing could be drastically reduced.
Magnetic resonance imaging and its closely related analogue nuclear magnetic resonance (NMR) spectroscopy are ideally suited to study drug dissolution phenomena at a local level within a dosage form for a number of reasons. Both MRI and NMR spectroscopic techniques are quantitative, non-invasive, chemical and nuclear specific techniques that can also be used to quantify molecular mass transport phenomena in the form of molecular diffusion and flow2. Both MRI and NMR phenomena rely on the fundamental nuclear properties of molecules in the form of nuclear spin angular momentum and in theory can be applied to any nucleus that contains an odd number of protons and/or neutrons. A nucleus with non-zero spin angular momentum has an associated magnetic moment vector that can be manipulated with electromagnetic radiation, in the form of radiofrequency pulses, to produce a time dependent signal that is electronically detectable. Fourier analysis of such a signal then leads to either an image or a spectrum depending on the particular magnetic resonance technique chosen by the operator. The vast majority of published MRI and NMR research concerns the humble hydrogen atom nucleus or proton (1H). The downside of magnetic resonance, unlike that of say infrared, Raman or X-ray techniques, is that it is an inherently insensitive technique meaning that obtaining an image or spectrum of sufficient signal-to-noise ratio can be time-consuming. This has the consequence that it becomes difficult to quantify a particular species’ concentration at low inherent levels (as is the case for many APIs in pharmaceutical formulations) in systems that evolve on timescales of a few seconds or even hours. In its simplest form the fundamental equation that describes magnetic resonance phenomena is given by the so-called Larmor equation: ω0 = γB0 (which for the most part is in the radiofrequency region of the electromagnetic spectrum) of a particular nucleus is proportional to the gyromagnetic ratio, γ, of that nucleus and the magnitude of the applied static magnetic field B0. The applied static field, which is typically between 2.35-11.7 Tesla and corresponds to resonance frequencies in the hundreds of mega-Hertz range, is usually achieved using physically large, expensive superconducting magnets. However, recent innovation has produced commercially available low cost low magnetic field (0.5 Tesla) bench-top MRI systems that can be used in pharmaceutical research3,4. In order to obtain spatially resolved information from a sample, i.e. an image, additional small magnetic field gradients G are applied to the sample under investigation which results in a modified Larmor equation of the form: ω0(r) = γ(B0 + G · r) is now dependent on the position r of a particular nucleus in the sample and the applied magnetic field gradient strength G. A thorough treatment of the basics of NMR and MRI theory can be found in Callaghan5 and a thorough review of the quantitative applications of MRI in pharmaceutical research can be found in Mantle6. One of the defining beauties of a magnetic resonance experiment is that is can, under the right conditions, reveal exactly what type of substance is present in a system, how much there is of it, and moreover, how it behaves with time.
Quantitative magnetic resonance imaging of gel layer formation in HPMC tablets
Recent developments in magnetic resonance techniques6 have shown that it is possible to obtain quantitative ‘nuclear spin-density’ maps of the dynamics of dissolution media ingress into hydroxypropylmethyl cellulose (HPMC) polymer at 390 micron spatial resolution in less than two minutes. HPMC is commonly used in controlled release delivery formulations as it forms a gel layer upon contact with water; the gel layer then limits the mass transport of API from the delivery device into the surrounding medium in a controlled fashion. The term ‘nuclear spin-density’ is often used in the magnetic resonance community and simply means how much of a particular substance we have within a specific volume element. It is extremely important to be able to quantify the local nuclear spin-density of a substance, whether it be the dissolution media itself or the API, during the dissolution process so that we may relate this behaviour to the in-line drug release curve that is the usual output from standard dissolution testing protocols. In addition to nuclear spin-density maps, it is also possible to obtain nuclear magnetic resonance spin-spin (T2) relaxation time maps and molecular self-diffusion coefficient maps (in units of m2s-1): T2-relaxation time maps essentially reflect how the dissolution medium, e.g. water, interacts with the swelling polymer and, if present, the API and formulation excipients; self-diffusion coefficient maps reflect the random mass transport of molecules within the delivery device and surrounding solution. Figure 1 (opposite) shows a series of one millimetre thick slice selective spin-density proton (1H) magnetic resonance images, 1H T2-relaxation maps and 1H water self-diffusion (D) maps taken at various points in time of a pure HPMC tablet undergoing dissolution / gelation in phosphate buffer solution (PBS, pH = 7.4) solution in a 25 millimetre diameter cylindrical tube phantom that can be incorporated into a commercial wide bore 400 MHz super – conducting MRI spectrometer7.
The 1H nuclear spin-density map in Figure 1 (I(a)) at t=0.5 hours shows the dry core of the tablet (black colour in the middle of image) surrounded by the dissolution medium (yellow colour). As time progresses (Figure 1 (b-c)), a gel layer that contains anywhere between 4 0-95 per cent water at any particular instant can be seen. However, what is not evident from the spin-density 1H MRI images alone is the boundary between the gel layer and bulk dissolution medium, i.e. free PBS solution. It is only by examining the complementary T2-relaxation time maps (Figure 1 (II)) and self-diffusion coefficient maps (Figure 1 (III)) that a clear distinction between the free PBS solution and outer edges of the gel layer can be observed. In addition to molecular selfdiffusion coefficient maps, which are essentially acquired under snapshot pseudo-equilibrium conditions, it is also possible to analyse the individual time series of the water concentration images using Fick’s laws of diffusion to extract macroscopic transport diffusivities. This type of combined quantitative information is extremely valuable for comparison with numerical models of such systems.
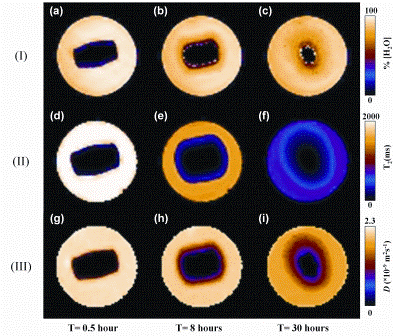
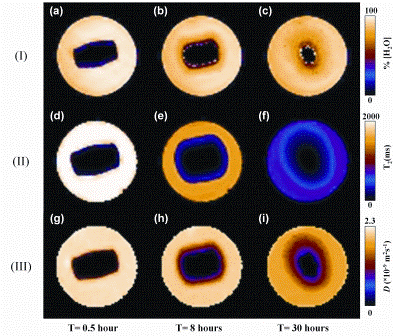
Figure 1: One millimetre thick, slice selective 1H images taken through a pure cylindrical HPMC tablet stood vertically at three different time points. (I)(a-c) 1H spin-density images that reflect water concentration. (II)(d-f) T2-relaxation time images that reflect the interaction of the water with the HPMC polymer. (II)(g-i) water self-diffusion coefficient maps. All images were acquired in less than two minutes in a field of view of 30 x 30 millimetres corresponding to an in-plane pixel resolution of 470 μm
MRI studies of Lescol®-XLTM dissolution in a USP-IV dissolution cell
Much early research activity focusing on MRI and tablet dissolution phenomena involved the use of in-house non-pharmacopeial compliant dissolution cells. However, recent developments in MRI hardware (and to some degree software) have allowed pharmacopeial compliant dissolution cells, e.g. United States Pharmacopeial (USP)-IV, to be incorporated into both high- and low-field MRI systems, operated under bio-relevant conditions. Figure 2 shows a series of quantitative 1H one millimetre thick slice selective MR images8 of a single Lescol®-XL tablet; a commercially available product that reduces cholesterol levels and thus slows the progression of heart disease. Here the tablet has been placed in a standard USP-IV dissolution cell under bio-relevant conditions of both simulated gastric fluid (SGF) for one hour followed thereafter using an in-line switch to fasted state simulated intestinal fluid (FaSSIF).
The three sets of three images show the following information: (I) absolute water concentration; (II) water T2-relaxation times; (III) the hydrodynamic VZ velocity field of water around the tablet during dissolution. The small dark dots present in the 1H spin-density images at t= 0.5 hours are associated with the nylon cage that holds the tablet in place during the dissolution experiment. The 1H spin-density images show an obvious gradient in water concentration as expected due to Fickian diffusion processes. It is also evident from images (b) and (c) that air bubbles are released from within the matrix during the dissolution process. In extreme cases9 this could cause a catastrophic cracking / breakage of the drug delivery matrix resulting in undesirable ‘dose dumping’ of the API. The T2 relaxation time maps shown in Figure 2 (II (d-f )) show the dynamics of the interaction of the dissolution media with the solid matrix and in particular highlight the evolution of the surface roughness of the outside of the tablet; such a parameter could play an important role in quantifying API release from model systems. The hydrodynamic VZ velocity field shown in Figure 2 (III (g-i)) initially shows an uneven distribution around the tablet but it is evident that this eventually settles down to form a more uniform annular VZ velocity field around the tablet indicating that the shear forces become more evenly distributed. Note that there is zero flow through the actual tablet. This type of information is almost impossible to obtain by any other noninvasive technique.
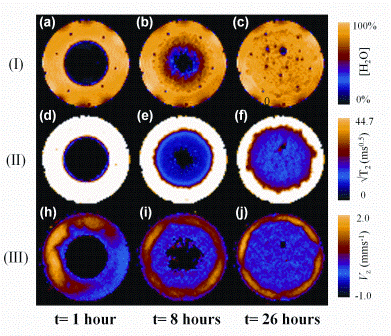
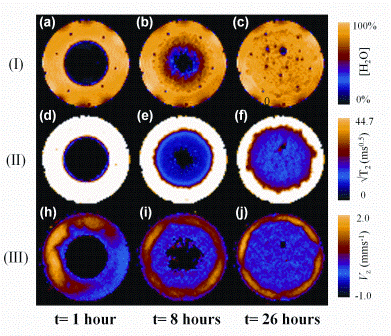
Figure 2: 1 mm thick, slice selective 1H images taken through a Lescol®-XL tablet lying in a horizontal orientation in a USP-IV dissolution apparatus operated at 37.5 degrees Celcius. Dissolution protocol: one hour of SGF dissolution medium followed by a in-line switch to FaSSIF thereafter for the duration of the experiment. Flow rate eight millilitres per minute-1. (I)(a-c) 1H spin-density images. (II)(d-f) T2-relaxation time images. (II)(g-i) Vz velocity images. All images were acquired in less than two minutes in a field of view of 24 x 24 millimetres corresponding to an in-plane pixel resolution of 375 μm
Direct imaging of the API during dissolution
Currently, an important experimental aim of applying magnetic resonance techniques to quantify pharmaceutical dissolution phenomena is to be able to simultaneously quantify both dissolution media ingress and API egress from a controlled delivery device with sufficient temporal and spatial resolution so that one could ultimately use this type of data to validate numerical simulations of the same system. However, direct imaging of the API is problematic in magnetic resonance for two main reasons: (1) the API represents a small fraction (by mass) of the whole delivery device thereby giving an inherently poor signal-to-noise ratio; (2) The API is ‘swamped’ by the huge excess of 1H signal from the dissolution media, which is essentially water. Because of (2) alternative active nuclei, e.g. carbon-13 (13C),fluorine-19 (19F) and nitrogen- 14(14N), that may or may not be present in the molecules of certain APIs, must be interrogated by magnetic resonance. Of these three nuclei, 19F is the only realistic proposition as the one per cent natural abundance of the 13C nucleus combined with its low gyromagentic ratio constant render it over three orders of magnitude worse in sensitivity when compared to that of the 1H nucleus. 14N may also be ruled out as it possesses a nuclear quadrupole moment which essentially renders it invisible to many magnetic resonance imaging experiments. Despite these drawbacks, one-dimensional 19F profile imaging of the API in Lescol®-XL is possible under pharmacopeial conditions8. The API dose in Lescol®-XL is 80 milligrams of Sodium fluvastatin, a small molecule which contains a single 19F nucleus. Figure 3 shows a series of one-dimensional 19F profiles of the mobilisation / dissolution of the fluvastatin API within the Lescol®-XL tablet during the complete dissolution test.
It should be stressed that no 19F signal is visible outside the boundaries primarily due to the fact that the dissolution medium is flowing and thus washes out the released API from the MRI active region of the apparatus. Inspection of Figure 3(a) shows that initially no 19F signal is visible in line with the fact that the API is initially in an immobile undissolved solid state form which is invisible to the magnetic resonance imaging method used in this experiment. The 19F signal only becomes visible when sufficient water has penetrated the Lescol®-XL tablet and a gel layer has started to form. Once a gel layer has formed, the API held within it dissolves and becomes MRI active. Figure 3(a) shows a steady increase in 19F signal reaching a maximum at around nine hours, followed by a steady decrease in 19F signal due to the combined effects of: (1) mass transport of the API out of the swollen matrix and into the surrounding (flowing) dissolution medium; (2) physical erosion of the tablet. It is important at all stages to try and relate the quantitative information obtained using magnetic resonance methods to those of traditional dissolution testing, i.e. drug release profiles. Figure 3(b) shows the integrated 19F-profile intensity plotted against time along with cumulative in-line ultraviolet visible (UV-vis) absorption spectroscopy data of the fluvastatin during dissolution. Figure 3(b) shows a good correlation between the constant drug release period between nine and 30 hours for both sets of independent data.
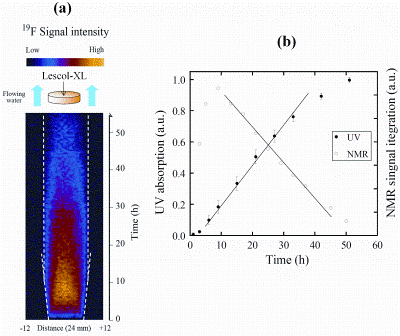
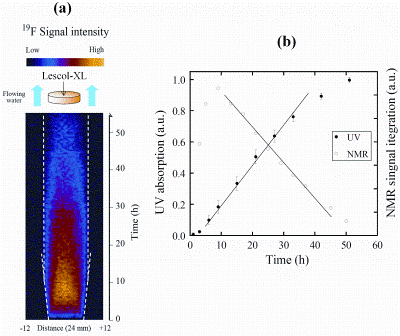
Figure 3: (a) A time series (vertical axis) of one-dimensional 19F spin-density profiles taken from the same Lescol®-XL tablet as that in Figure 2. (b) A comparison of the integrated 19F-profile intensity with the in line UV visible absorption spectra from the same tablet
Summary remarks
Due to the advances in magnetic resonance imaging hardware and software, it is now possible to routinely use these techniques in a quantitative fashion to study pharmaceutical tablet dissolution phenomena. The data that can be currently gleaned from such methods is summarised as follows:
- Time dependent water concentration profiles from MRI data can be analysed using Fick’s laws, along with appropriate mass balances and boundary conditions, to produce transport diffusivities of dissolution media ingress and API egress
- T2-relaxation time maps can be used to give unambiguous information regarding gel layer boundaries of controlled release devices but may also be used to give qualitative insights into the physical interactions of the dissolution media with the API and matrix polymer formulation
- Molecular self-diffusivities can be extracted from diffusion weighted MRI data
- Hydrodynamic velocity fields can be calculated directly from MRI velocity data thereby facilitating local shear stress calculations around/at the surface of a dosage form
The application of MRI and NMR to pharma – ceutical research has come a long way since its first applications back in the early 1990s. However a number of challenges still remain. For example, we are only beginning to look directly at APIs in-vitro, in real systems under biorelevant conditions and much further work is required to have a deeper understanding of in-vivo/in-vitro correlations. MRI and NMR will likely always struggle with sensitivity issues but new techniques such as compressed sensing10 (CS) MR signal acquisition and hyperpolarised nuclear species11 could be used in this area to alleviate such problems.
Acknowledgements
The author wishes to thank the following people and pharmaceutical companies for their scientific input and financial support: Dr Ya Ying Chen, Dr Les Hughes and AstraZeneca; Dr Qilei Zhang, Dr Paolo Avalle and Merck Sharp & Dohme and Professor Lynn F. Gladden for her continued support.
References
1. Y.Perrie, T. Rades, (2010). Pharmaceutics – Drug delivery and targeting, 1st ed. pharmaceutical press, London
2. M.D. Mantle & A.J. Sederman, Dynamic MRI in chemical process and reaction engineering. Progress in Nuclear Magnetic Resonance Spectroscopy, 43, (2003), 3-60
3. K.P. Nott, Magnetic resonance imaging of tablet dissolution. European Journal of Pharmaceutics and Biopharmaceutics 74(1), (2010), 78-83
4. V. Malaterre, H. Metz, J. Ogorka, R. Gurny, N. Loggia, K. Mader, Benchtop-magnetic resonance imaging (BT-MRI) characterization of push-pull osmotic controlled release systems. Journal of Controlled Release 133(1), (2009), 31-36
5. P.T. Callaghan, Principles of Nuclear Magnetic Resonance Microscopy, Oxford University Press, New York, 1991
6. M.D. Mantle, Quantitative magnetic resonance micro-imaging methods for pharmaceutical research, International Journal of Pharmaceutics, 417, (2011), 173-195.
7. Y.Y. Chen, L.P. Hughes, L.F. Gladden, M.D. Mantle, Quantitative Ultra-Fast MRI of HPMC Swelling and Dissolution. Journal of Pharmaceutical Sciences 99(8) (2010) 3462-3472
8. Q. Zhang, P. Avalle, L. Gladden, M. Mantle, In vitro quantitative 1H and 19F nuclear magnetic resonance spectroscopy and imaging studies of fluvastatin in Lescol®-XL tablets in a USP-IV dissolution cell. Journal of Controlled Release, 156(3), 2011, 345-354
9. J.H.P. Collins, L.F. Gladden, I.J. Hardy, M.D. Mantle, Characterizing the evolution of porosity during controlled drug release. Applied Magnetic Resonance 32(1-2) (2007) 185-204
10. M. Lustig; D. Donoho; J. M. Pauly, Magnetic Resonance in Medicine 2007, 58, (6), 1182-1195
11. J. Wolbera, F. Ellnera, B. Fridlunda, A. Grama, H. Johannessona, G. Hanssona, L.H. Hanssona, M.H. Lerchea, S. M(anssonb, R. Servina, M. Thaninga,K. Golmana, J.H. Ardenkjær-Larsena, Generating highly polarized nuclear spins in solution using dynamic nuclear polarization, Nuclear Instruments and Methods in Physics Research A 526, (2004), 173–181