Preparing biocompatible materials for non-permanent medical devices
Posted: 22 October 2012 | | No comments yet
Biodegradable polymers comprise an important class of biomaterials due to their ability to satisfy short-term requirements for medical applications where a permanent implant is not required. However, current biodegradable polymers suffer from undesirable chemical properties that lead to improper elimination from the body and potentially toxic by-products. Additionally, medical polymers cause adverse biological responses at the material-body interface and therefore require some functionality to regulate such processes. To overcome problems such as processability and solubility issues, we describe our approach to synthesising new classes of biodegradable polymers with desirable structural features that exhibit good solubility, tunable degradation and non-toxic by-products. Additionally, these polymers are functionalised for release of the therapeutic agent, nitric oxide (NO), which serves to regulate initial and long-term biological responses.
Two different classes of modified polymer are presented; the first is based on poly(lactic-coglycolic acid) (PLGH) and the second on dextran. We present the NO release profiles associated with several polymer derivatives of both classes along with their degradation timelines. Overall, the PLGH derivatives are processable as films or fibres and show promise in wound healing and tissue engineering applications while the dextran materials are feasible as prodrugs in the cardiovascular system.
Biodegradable polymers comprise an important class of biomaterials due to their ability to satisfy short-term requirements for medical applications where a permanent implant is not required. However, current biodegradable polymers suffer from undesirable chemical properties that lead to improper elimination from the body and potentially toxic by-products. Additionally, medical polymers cause adverse biological responses at the material-body interface and therefore require some functionality to regulate such processes. To overcome problems such as processability and solubility issues, we describe our approach to synthesising new classes of biodegradable polymers with desirable structural features that exhibit good solubility, tunable degradation and non-toxic by-products. Additionally, these polymers are functionalised for release of the therapeutic agent, nitric oxide (NO), which serves to regulate initial and long-term biological responses.Two different classes of modified polymer are presented; the first is based on poly(lactic-coglycolic acid) (PLGH) and the second on dextran. We present the NO release profiles associated with several polymer derivatives of both classes along with their degradation timelines. Overall, the PLGH derivatives are processable as films or fibres and show promise in wound healing and tissue engineering applications while the dextran materials are feasible as prodrugs in the cardiovascular system.
Biodegradable polymers comprise an important class of biomaterials due to their ability to satisfy short-term requirements for medical applications where a permanent implant is not required. However, current biodegradable polymers suffer from undesirable chemical properties that lead to improper elimination from the body and potentially toxic by-products. Additionally, medical polymers cause adverse biological responses at the material-body interface and therefore require some functionality to regulate such processes. To overcome problems such as processability and solubility issues, we describe our approach to synthesising new classes of biodegradable polymers with desirable structural features that exhibit good solubility, tunable degradation and non-toxic by-products. Additionally, these polymers are functionalised for release of the therapeutic agent, nitric oxide (NO), which serves to regulate initial and long-term biological responses.
Two different classes of modified polymer are presented; the first is based on poly(lactic-coglycolic acid) (PLGH) and the second on dextran. We present the NO release profiles associated with several polymer derivatives of both classes along with their degradation timelines. Overall, the PLGH derivatives are processable as films or fibres and show promise in wound healing and tissue engineering applications while the dextran materials are feasible as prodrugs in the cardiovascular system.
Polymers, naturally-occurring and synthetic, represent an important class of biomedical materials that are used extensively in applications ranging from implants to externally applied devices. The use of natural polymers such as cotton, cellulose and collagen for medical purposes dates back centuries. The very first reported synthetic polymer of medical relevance is poly(methyl methacrylate) (PMMA) which was used to make eye lens implants (intraocular lenses) in 1949 by British ophthalmologist Sir Nicholas Harold Ridley1. Successively, a number of polymeric materials such as poly(ethylene terephthalate), poly(dimethylsiloxane), poly(tetrafluoro – ethylene) and polyurethane were used for various medical applications2-5. As indicated in Figure 1, several different biostable synthetic polymers are currently processed for use as permanent implants critical to human health, including heart valves, joint and bone replacements, and stents. However, a whole host of short-term medical applications do not require a permanent device, such as bone and tissue engineering, cell growth scaffolds, sutures, bandages and drug delivery vesicles. These short-term applications require several critical polymer features, including the material lifetime and mechanical support. Therefore, the advent of biodegradable polymers marked a significant change in the biomaterials community for temporary biomedical applications.
Biodegradable polymers can provide sufficient protection against proteolytic degradation in drug delivery systems or necessary mechanical supports in orthopaedic applications. Once the short-term function of the material is satisfied, the polymer can finally degrade hydrolytically or enzymatically in vivo into non-toxic products for complete elimination from the body. Figure 1 additionally highlights applications where biodegradable polymers are critical to sustain function until the body can restore natural function. For instance, the material may persist until its drug payload is delivered or the material may offer mechanical support to allow for cell generation until tissue growth is sufficient. As such, it is critical that the lifetime of the material match that of the intended application.
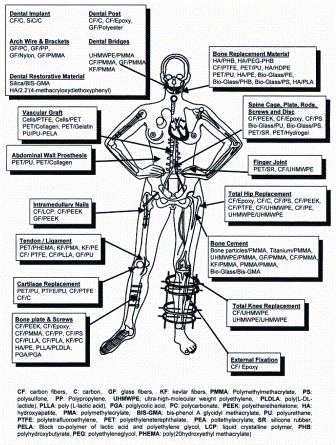
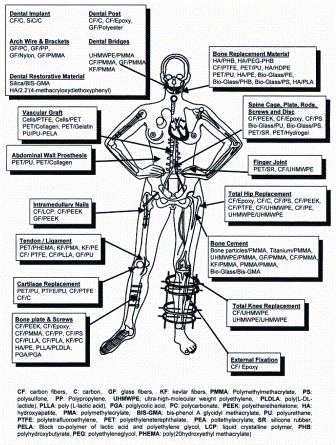
Figure 1: A variety of polymeric biomaterials are critical to maintain different biological functions within the human body. Reproduced with permission12
Commonly used synthetic biodegradable polymers include polyesters, polyanhydrides, polyacetals, polyketals, polyorthoesters, polycarbonates, polyurethanes, poly – phosphazenes, polyphosphoesters and polydioxanones. The very first clinically investigated biodegradable polymer was poly(glycolic acid) (PGA), which was approved by both the United States Pharmacopoeia (USP) and the European Pharmacopoeia (EP) in 1970 towards the development of a synthetic absorbable surgical suture, DEXONTM6. The majority of these synthetic polymers are credited with their ease of preparation, good processability, tailorable and predictable degradation profiles and sufficient mechanical strength to support the required application. Moreover, physical and chemical properties can be conveniently varied by tuning the monomer substituents and compositions during polymerisation or post-polymerisation modifications. However, the degradation products tend to exhibit cytotoxic and hydrophobic properties, preventing sufficient elimination of by-products from the material site. As a result, undesired swelling and inflammatory responses are serious drawbacks associated with these polymers.
To overcome toxicity issues associated with biodegradable polymers, it is useful to consider naturally occurring polymers, such as those that exist in the body. Polymers of natural origin constitute a very important class of biomedical materials because of their biocompatibility, non-cytotoxicity, similarity to the extracellular matrix and good biological performances in general7. Examples include proteins (collagen, fibrin), polyamino acids (polyglutamic acid, polylysine), and polysaccharides (hyaluronic acid, chondroitin sulphate, chitin, chitosan, alginate, pullulan and dextran). However, poor processability and solubility issues associated with these natural polymers have prevented their widespread commercial use. Since these materials possess great promise due to their natural biofunctionality, significant scientific efforts are currently underway to make use of these materials. Approaches include creating either composite materials, where the natural polymer is blended with another processable synthetic polymer, or copolymers consisting of chemically linked natural and synthetic polymers8-11. To provide a comp – rehensive review of all currently available biodegradable synthetic and natural polymers used for biomedical applications would be beyond the scope of this article and many reviews on the topic have been recently published12-16.
Biological responses to biomaterials
Although biodegradable biomaterials have become a promising strategy for various biomedical applications, fatal complications and device failures are routinely encountered from the powerful extracellular influences and molecular interactions at the material-biology interface17,18. Implanted biomaterials, both biostable and biodegradable, in general nitiate a series of tissue responses as well as elicit non-specific protein adsorption, which is accompanied by denaturation and changes in protein conformation. These protein changes initiate cell-signalling processes that lead to platelet adhesion, followed by systemic inflammation and blood clot formation at the material site19. A schematic illustration of this cascade of events is illustrated in Figure 2. Moreover, in the case of biostable materials, the persistence of the material results in chronic macrophage aggregation, thus triggering undesired immune responses and adversely affecting the longevity of the implant20. Toxicity issues associated with by-products of bio – degradable materials also lead to complications. Overall, severe limitations are experienced towards the end application of implanted materials due to biocompatibility issues that ultimately lead to device failure.
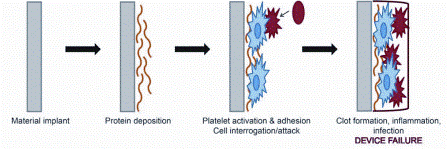
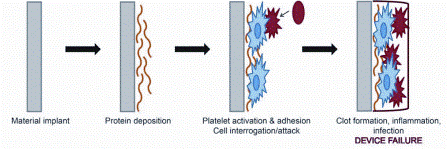
Figure 2: The cascade of events leading to blood clot formation on the passive biomaterial surface consists largely of protein and platelet deposition, followed by protein conformational changes to promote thrombus formation
Need for bioresponsive biomedical materials
The adverse biological responses described above are typical among passive biomaterials that exist simply to perform a basic function. For example, a biostable polymer that is processed into a coronary stent serves the purpose of holding open coronary arteries, thus preventing constriction of blood flow to the heart. The above-mentioned processes of blood clot formation and inflammation that arise at the material-blood interface will prevent the stent from performing its basic function of admitting blood flow, resulting in device failure. To overcome the current constraints associated with such existing passive biomedical materials, next generation materials should control bioresponses to maintain proper function at the material site. In addition to the stent performing its function of regulating blood flow, functionality must be included to regulate adhesion of biological factors to the material surface to prevent device malfunction. Major approaches to creating functional biomaterials include surface modifications to control adsorption processes or the release of drugs that regulate biological activities, such as heparin21. In general, it is critical that the functional biomaterial be integrated within the body such that undesired biological processes are prevented, while others, such as wound healing and appropriate cell growth, are promoted. Essentially, it is critical to the function of the device, and ultimately patient safety, that homeostasis be maintained despite disruption due to biomaterial incorporation for both short and long-term applications.
Mimicking the natural endothelium
To our knowledge, the natural endothelium is the best thromboresistant surface ever known, thus a material that can mimic the properties of endothelial cells would be an ideal material for blood-contacting applications22. The natural endothelium produces nitric oxide (NO), which is one of the factors responsible for promoting and modulating hemostasis and regulating vascular tone within the body23,24. Moreover, NO, a bioagent and highly reactive free radical, is involved in many physiological processes including platelet inhibition, monocyte inactivation and anti-inflammatory responses (Figure 3)25-27. Researchers have also shown NO as an effector in wound healing mechanisms28,29 and as an important regulator of angiogenesis and revascularisation30,31. By harnessing all of the natural therapeutic properties exhibited by NO, a truly multifunctional material can be created for ideal incorporation within the body toward the development of materials for a range of biomedical applications.
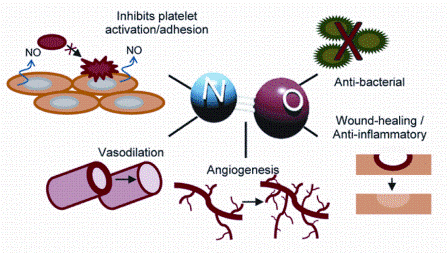
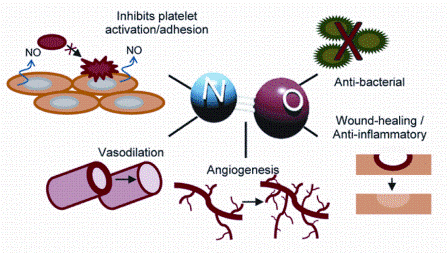
Figure 3: Nitric oxide exhibits multiple therapeutic properties and is involved in a variety of biological activities
Due to the reactive nature of the NO radical, NO must be stored as a stable NO donor within a system until delivery is initiated. Many different NO donors have been reported, with S-nitrosothiols (RSNOs) and N-diazeniumdiolates comprising the majority of the NO prodrug and material literature due to their straightforward synthesis and versatile mechanisms of decomposition32. Nitric oxide donor-containing polymers have gained much attention since the first report of N-diazeniumdiolate incorporation within polymers in 199633. Major approaches currently include (a) the preparation of composite materials by blending preformed NO donors within polymer matrices and (b) the covalent attachment of NO donors onto polymer backbones or pendant groups34-37. The incorporation of NO releasing capabilities within synthetic polymers has been demonstrated to enhance cell proliferation and prevent platelet adhesion and thrombus formation compared to control samples, thus providing a very promising strategy towards the development of biomimetic vascular grafts22,38. Additionally, NO releasing polymers have demonstrated excellent anti-bacterial properties39-41.
NO releasing degradable biomaterials: our approach
It is important to note that, within the field of NO releasing polymers, very few biodegradable materials have been reported to date, with the majority of the methods employing N-diazeniumdiolate donor groups35. N-diazeniumdiolates have gained popularity due to their ease of synthesis (exposure of amine sites to NO gas) and straightforward route of decomposition (H+ and heat initiated). However, RSNOs exhibit a more versatile synthesis (nitrosation of a thiol site) and decomposition, making these donors highly tunable for different applications. Our approach involves covalently incorporating S-nitrosothiol moieties within biodegradable polymer structures. Thiol sites are nitrosated whenever a source of NO+ is present; therefore, NO loading can occur under aqueous (HNO2)42, organic solvent conditions (t-butyl nitrite)43 or within the solid phase (exposure to oxygenated NO gas)44. The various nitrosating conditions allow for the material to be selectively loaded, subject to the material requirements and end applications45. Material nitrosation is typically accomplished by exposing a solid thiol-containing material to nitrous acid46-51. However, the harsh conditions associated with this process may compromise the integrity of the polymer. Additionally, nitrosation in the solid phase may result in less efficient NO loading as well as residual nitrite being trapped in the material system. Depending upon the RSNO decomposition mechanism employed, the reduction of residual nitrite will contribute to the directly detected NO45. To overcome the potential problems associated with solid-phase nitrous acid nitrosation, we instead expose our material in an organic solution to t-butyl nitrite. The use of volatile t-butyl nitrite ensures complete removal of the nitrosating agent under vacuum and the non-aqueous conditions maintain the polymer integrity52. The resulting nitrosated polymer can then be recovered for processing.
S-nitrosothiols release NO via the homolytic cleavage of the S-N bond through a variety of pathways, including pH, heat, light and metal ion initiated decomposition53,54. Because of the many RSNO decomposition mechanisms, RSNO containing polymers can be tailored to deliver NO over a wide range of conditions, including phototherapeutic treatments. Additionally, RSNOs are endogenous sources of NO transport and delivery within the body and are non-toxic in nature. Overall, the rich chemistry associated with the formation and decomposition of RSNO donors opens up many possibilities for their synthesis and final application as NO releasing materials.
NO releasing biodegradable polymers for wound healing and tissue engineering
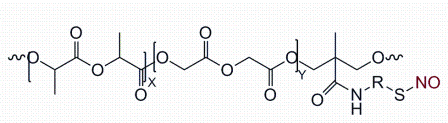
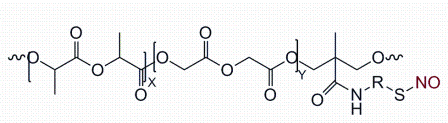
Figure 4: The PLGH polymer was modified to contain different pendant groups that are available for NO loading where R represents cysteamine, cysteine, homocysteine or other structural analogues
The first generation materials (Figure 4) we prepared are based on a biodegradable polyester backbone prepared from L-lactide (LA), glycolide (GL) and 2,2’-bis(hydroxymethyl propionic acid) (HMPA)52,55. The resulting poly(lactic-co-glycolic-co-hydroxymethyl pro – pionic acid) (PLGH) polymer was functionalised by covalently conjugating a number of thiol amino acids (cysteine, homocysteine, cyste – amine) to the polymer to generate a series of thiolated polymer derivatives. Finally, the pendant thiol terminals were nitrosated under anhydrous conditions to generate stable RSNOs for the storage and controlled delivery of NO. We successfully prepared polymer thin films as well as electrospun nanofibres from the corresponding polymer solutions56. Fibres of the modified polymers exhibited smooth, bead free morph – ologies with diameters averaging between 200 and 410 nanometres (Figure 5). The pro cessability of these materials to generate nanofibres is promising because nanofibres have been shown to mimic the behaviour of the extracellular matrix, thereby providing a useful tool for making tissue engineering nanoscaffolds57.
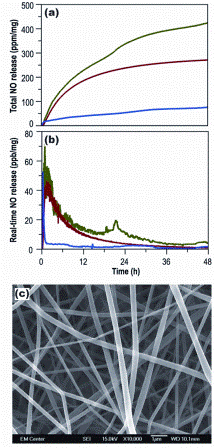
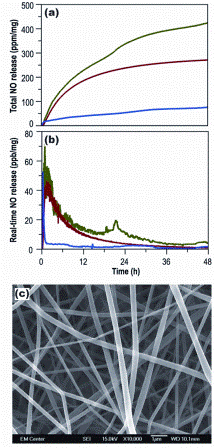
Figure 5: (a) Total and (b) real-time NO release curves associated with the S-nitrosated PLGH derivatives (cysteamine in green, cysteine in red, homocysteine in blue) under physiologicalconditions where the polymers were processed as thin films (materials processed as nanofibres demonstrated similar release profiles). (c) Representative SEM image of S-nitrosated PLGHcysteamine processed as electrospun nanofibres
Real-time NO release profiles from the different polymer forms were evaluated using highly selective and sensitive ozone-based chemiluminescence NO analysers. Repre – sentative real-time and total NO release profiles are shown in Figure 5 on page 74, demonstrating controlled and extended NO release over 48 hours. All polymers provided stable NO storage as the RSNO groups remained intact even when subjected to high voltage during the electrospinning process. In an earlier attempt to create NO releasing electrospun nanofibres58, polymers containing composite donor blends released their NO payload within a period of minutes. However, our materials provided continuous and sustained release of NO over a period of days, which is a more practical timeframe of therapeutic action for biomedical applications.
In vitro degradation of the polymers was evaluated gravimetrically under physiological conditions (37°C, pH 7.4). Nearly 20 per cent weight loss was observed in the first week for all polymers, followed by a slow but linear rate of degradation for the subsequent weeks. Linear extrapolation of the decomposition rate predicts complete degradation of the material over nearly 40, 30 and 20 weeks for the cysteamine, cysteine and homocysteine derivatives, respectively. Degradation profiles of all S-nitrosated polymer derivatives were found to have a similar kinetics to that of the parent thiol derivative.
A preliminary biocompatibility evaluation using the ISO 10993-5 cytotoxicity elution method (ISO-10993-5: Biological Evaluation of Medical Devices, Part 5: Tests for In Vitro Cytotoxicity, performed at NAMSA in Northwood, OH, USA) showed no cytotoxicity associated with any of these materials, fulfilling another critical requirement for use in biomedical applications. Surface wettability studies using static water contact angle presented an overall moderate hydrophilic nature for thiolated as well as S-nitrosated polymers (70 – 77 degrees). The moderate wettability associated with these polymers is favourable for cell attachment and proliferation, and is not a property exhibited by the majority of other synthetic polymers.
Initial antimicrobial evaluations were performed using Acinetobacter baumannii (Ab), a species of pathogenic bacteria of particular interest to the medical community because of its multidrug-resistivity, which results in the death of tens of thousands of patients each year in the United States alone59. Moreover, this bacterial infection is prevalent in troops wounded in Afghanistan and Iraq60. Our treatment of S-nitrosated PLGH-cysteamine with Ab for two hours at 37°C in buffer (pH 7.4) resulted in a 96 per cent reduction in the bacterial counts (treatment dosage of 0.0035 mmol of NO). This efficiency is higher than any other reported NO delivery material to date; previous studies using NO releasing sol-gel derived xerogels in an adhesion model have shown a maximum bacterial reduction of 70 – 80 per cent61,62. Further studies are underway to understand the dose-response relationship of the NO releasing materials in various fabrication forms.
Overall, we have demonstrated the capability of these NO releasing biodegradable polymers to prevent initial bioresponses such as antimicrobial activity. Furthermore, their predictable biodegradable nature and processability make for a versatile class of materials for wound healing and tissue engineering applications. Detailed evaluations of these materials targeting biomedical applications are in progress, including in vitro platelet and protein deposition studies, determination of the real-time degradation products, and in vivo evaluation of their performance as wound healing patches.
NO releasing natural polymers as prodrugs
In addition to the thromboresistive capabilities discussed previously, NO exerts a variety of vasoprotective effects63 and has been found to be very effective in the treatment of cancer64. However, the exact potential of this multifaceted therapeutic agent is still to be exploited in treatments clinically because of non-specific and targeted delivery issues. We have recently developed a series of enzymatically degradable NO releasing dextran derivatives as stable prodrugs to treat cardiovascular disease. Dextran, a natural polysaccharide, is used as a blood plasma volume expander and approved by the FDA as Generally Recognised As Safe (GRAS) for food and drug applications.
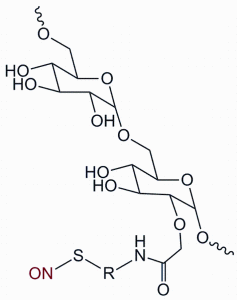
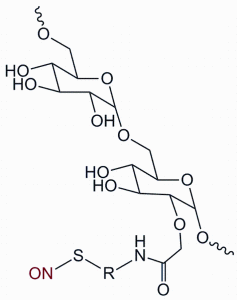
Figure 6: The polymer dextran was modified to contain different pendant groups that are available for NO loading where R represents cysteamine, cysteine or other structural analogues
We successfully derivatised the dextran backbone with a number of thiol derivatives including cysteine and cysteamine (Figure 6) where the very specific conjugation chemistries employed resulted in stable Snitrosothiol moieties for NO storage and delivery. This resulted in a new class of functional macro molecules with exceptional NO release capabilities in arterial blood conditions (pH 7.38 – 7.41) followed by in vivo enzymatic degradation via intestinal dextranase on the order of days. Nearly complete NO recovery was attained from these materials under physiological conditions within six hours (Figure 7).
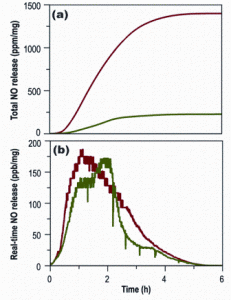
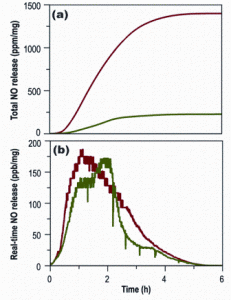
Figure 7: (a) Total and (b) real-time NO release curves from S-nitrosated dextran derivatives under physiological conditions (cysteamine in green, cysteine in red)
This NO release rate is comparable to the blood circulatory period for dextran with similar molecular weights. Consequently, these derivatives are devised to release the majority of the available NO during the blood circulation period before reaching the liver and spleen, where they are finally metabolised by dextranase. In summary, we anticipate that these NO releasing S-nitrosated dextran derivatives will serve as a safe and cost effective prodrug treatment for a variety of cardiovascular disorders including coronary artery disease. Detailed biological evaluations of these materials are currently underway including dose-response studies for prodrug application of these materials.
Conclusions
Overall, the modified PLGH and dextran biodegradable polymers reported herein demonstrate great promise as biomaterials. We exhibit fine-tuned control over the polymer structure by way of synthetic conditions as well as the NO loading process via nitrosation. The final structures of the nitrosated derivatives allow for different degradation and NO release timelines toward different short-term biological applications. For the PLGH and dextran derivatives, the lifetime of NO release and biodegradation make these materials appro – priate for wound healing and prodrug applications, respectively. We demonstrate that these materials are processable, thus overcoming one of the major drawbacks currently associated with biodegradable materials as these materials can be made available in different forms. Toward the development of functional biodegradable materials, these polymers show promise due to their short-term and controllable release of a therapeutic agent and their predictable degradation into non-toxic by-products.
Acknowledgements
The authors acknowledge financial support for this research from Colorado State University and the Department of Defense Congressionally Directed Medical Research Program (DOD CDMRP). This research was supported by funds from the Boettcher Foundation’s Webb-Waring Biomedical Research Program.
References
1. D. G. Castner and B. D. Ratner, Surface Science, 2002, 500, 28-60
2. Z. Voldřrich, Z. Tománek, J. Vacík and J. Kopečcek, Journal of Biomedical Materials Research, 1975, 9, 675-685
3. E. Ascer, F. J. Veith, S. K. Gupta, G. Krasowski, R. H. Samson, L. A. Scher, S. A. White-Flores and S. Sprayregen, Journal of Cardiovascular Surgery, 1985, 26, 468-472
4. A. Colas and J. Curtis, Biomaterial Science: An Introduction to Materials in Medicine, Elsevier, California, USA, 2004
5. M. Szycher, J. C. Griffin, J. L. Williams, J. P. McMenamy and D. Stagg, Journal of Biomaterials Applications, 1987, 2, 290-313
6. J. B. Herrmann, R. J. Kelly and G. A. Higgins, Archives of Surgery, 1970, 100, 486-490
7. J. F. Mano, G. A. Silva, H. S. Azevedo, P. B. Malafaya, R. A. Sousa, S. S. Silva, L. F. Boesel, J. M. Oliveira, T. C. Santos, A. P. Marques, N. M. Neves and R. L. Reis, Journal of the Royal Society Interface, 2007, 4, 999-1030
8. J. Jiang, X. D. Pan, J. X. Cao, J. L. Jiang, D. B. Hua and X. L. Zhu, International Journal of Biological Macromolecules, 2012, 50, 586-590
9. Q. Yuan, R. Venkatasubramanian, S. Hein and R. D. K. Misra, Acta Biomaterialia, 2008, 4, 1024-1037
10. K. Y. Lee and D. J. Mooney, Progress in Polymer Science, 2012, 37, 106-126
11. K. Nagahama, Y. Mori, Y. Ohya and T. Ouchi, Biomacromolecules, 2007, 8, 2135-2141
12. S. Ramakrishna, J. Mayer, E. Wintermantel and K. W. Leong, Composites Science and Technology, 2001, 61, 1189-1224
13. B. D. Ulery, L. S. Nair and C. T. Laurencin, Journal of Polymer Science, Part B: Polymer Physics, 2011, 49, 832-864
14. L. S. Nair and C. T. Laurencin, Progress in Polymer Science, 2007, 32, 762-798
15. R. J. Kroeze, M. N. Helder, L. E. Govaert and T. H. Smit, Materials, 2009, 2, 833-856
16. J. C. Middleton and A. J. Tipton, Biomaterials, 2000, 21, 2335-2346
17. A. Lendlein, A. T. Neffe, B. F. Pierce and J. Vienken, International Journal of Artificial Organs, 2011, 34, 71-75
18. E. S. Place, N. D. Evans and M. M. Stevens, Nature Materials, 2009, 8, 457-470
19. J. M. Anderson, Annual Review of Materials Research, 2001, 31, 81-110
20. T. N. Salthouse, Journal of Biomedical Materials Research, 1984, 18, 395-401
21. S. W. Kim and H. Jacobs, Blood Purification, 1996, 14, 357-372
22. M. M. Reynolds and G. M. Annich, Organogenesis, 2011, 7, 42-49
23. R. F. Furchgott, Angewandte Chemie, International Edition, 1999, 38, 1870-1880
24. L. J. Ignarro, G. M. Buga, K. S. Wood, R. E. Byrns and G. Chaudhuri, Proc. Natl. Acad. Sci. USA, 1987, 84, 9265- 9269
25. B. G. Hill, B. P. Dranka, S. M. Bailey, J. R. Lancaster and V. M. Darley-Usmar, Journal of Biological Chemistry, 2010, 285, 19699-19704
26. P. B. Massion, O. Feron, C. Dessy and J. L. Balligand, Circulation Research, 2003, 93, 388-398
27. M. W. Radomski, Annals of Medicine, 1995, 27, 321-329
28. J. S. Isenberg, L. A. Ridnour, M. G. Espey, D. A. Wink and D. D. Roberts, Microsurgery, 2005, 25, 442-451
29. M. Rizk, M. B. Witte and A. Barbul, World Journal of Surgery, 2004, 28, 301-306
30. D. L. Contreras, H. V. Robles, E. Romo, A. Rios and B. Escalante, Pharmacology & Therapeutics, 2006, 112, 553-563
31. O. Gallo, E. Masini, L. Morbidelli, A. Franchi, I. Fini-Storchi, W. A. Vergari and M. Ziche, Journal of the National Cancer Institute, 1998, 90, 587-596
32. P. G. Wang, M. Xian, X. P. Tang, X. J. Wu, Z. Wen, T. W. Cai and A. J. Janczuk, Chemical Reviews, 2002, 102, 1091-1134
33. D. J. Smith, D. Chakravarthy, S. Pulfer, M. L. Simmons, J. A. Hrabie, M. L. Citro, J. E. Saavedra, K. M. Davies, T. C. Hutsell, D. L. Mooradian, S. R. Hanson and L. K. Keefer, Journal of Medicinal Chemistry, 1996, 39, 1148-1156
34. M. C. Jen, M. C. Serrano, R. van Lith and G. A. Ameer, Advanced Functional Materials, 2012, 22, 239-260
35. D. A. Riccio and M. H. Schoenfisch, Chemical Society Reviews, 2012, 41, 3731-3741
36. V. N. Varu, N. D. Tsihlis and M. R. Kibbe, Vascular and Endovascular Surgery, 2009, 43, 121-131
37. M. C. Frost, M. M. Reynolds and M. E. Meyerhoff, Biomaterials, 2005, 26, 1685-1693
38. S. Verma and P. A. Marsden, New England Journal of Medicine, 2005, 353, 730-731
39. K. P. Dobmeier and M. H. Schoenfisch, Biomacromolecules, 2004, 5, 2493-2495
40. J. Holt, B. Hertzberg, P. Weinhold, W. Storm, M. Schoenfisch and L. Dahners, Journal of Orthopaedic Trauma, 2011, 25, 432-437
41. E. M. Hetrick and M. H. Schoenfisch, Chemical Society Reviews, 2006, 35, 780-789
42. D. M. Byler, D. K. Gosser and H. Susi, Journal of Agricultural and Food Chemistry, 1983, 31, 523-527
43. M. J. Crookes and D. L. H. Williams, J. Chem. Soc. Perkin Trans. 2, 1989, 1319-1322
44. V. G. Kharitonov, A. R. Sundquist and V. S. Sharma, Journal of Biological Chemistry, 1995, 270, 28158-28164
45. J. M. Joslin and M. M. Reynolds, ACS Applied Materials & Interfaces, 2012, 4, 1126-1133
46. N. A. Stasko, T. H. Fischer and M. H. Schoenfisch, Biomacromolecules, 2008, 9, 834-841
47. D. A. Riccio, K. P. Dobmeier, E. M. Hetrick, B. J. Privett, H. S. Paul and M. H. Schoenfisch, Biomaterials, 2009, 30, 4494-4502
48. P. N. Coneski, K. S. Rao and M. H. Schoenfisch, Biomacromolecules, 2010, 11, 3208-3215
49. A. B. Seabra, D. Martins, M. Simoes, R. da Silva, M. Brocchi and M. G. de Oliveira, Artificial Organs, 2010, 34, E204-E214
50. P. N. Coneski and M. H. Schoenfisch, Polymer Chemistry, 2011, 2, 906-913
51. D. A. Riccio, P. N. Coneski, S. P. Nichols, A. D. Broadnax and M. H. Schoenfisch, ACS Applied Materials & Interfaces, 2012, 4, 796-804
52. V. B. Damodaran, J. M. Joslin, K. A. Wold, S. M. Lantvit and M. M. Reynolds, Journal of Materials Chemistry, 2012, 22, 5990-6001
53. K. Szacilowski and Z. Stasicka, Progress in Reaction Kinetics and Mechanism, 2001, 26, 1-58
54. D. L. H. Williams, Acc. Chem. Res., 1999, 32, 869-876
55. V. B. Damodaran and M. M. Reynolds, Journal of Materials Chemistry, 2011, 21, 5870-5872
56. K. A. Wold, V. B. Damodaran, L. A. Suazo, R. A. Bowen and M. M. Reynolds, ACS Applied Materials & Interfaces, 2012, 4, 3022-3030
57. Z. W. Ma, M. Kotaki, R. Inai and S. Ramakrishna, Tissue Engineering, 2005, 11, 101-109
58. P. N. Coneski, J. A. Nash and M. H. Schoenfisch, ACS Applied Materials & Interfaces, 2011, 3, 426-432
59. L. Dijkshoorn, A. Nemec and H. Seifert, Nature Reviews Microbiology, 2007, 5, 939-951
60. J. H. Calhoun, C. K. Murray and M. M. Manring, Clinical Orthopaedics and Related Research, 2008, 466, 1356-1362
61. B. J. Nablo, T. Y. Chen and M. H. Schoenfisch, Journal of the American Chemical Society, 2001, 123, 9712-9713
62. B. J. Nablo, A. R. Rothrock and M. H. Schoenfisch, Biomaterials, 2005, 26, 917-924
63. R. De Caterina, P. Libby, H.-B. Peng, V. J. Thannickal, T. B. Rajavashisth, M. A. Gimbrone, Jr., W. S. Shin and J. K. Liao, Journal of Clinical Investigation, 1995, 96, 60-68
64. D. Hirst and T. Robson, Journal of Pharmacy and Pharmacology, 2007, 59, 3-13
About the authors
Dr. Vinod B. Damodaran, a Research Scientist at Colorado State University, first began his research career as a pharmaceutical chemist with Merck KGAa. He received his doctorate in Chemical and Process Engineering from University of Canterbury, New Zealand under Professor Conan J. Fee in 2010. During his doctoral studies, Dr. Damodaran was the recipient of a University of Canterbury doctoral scholarship (2006 – 2009), a BioRad-BIC international travel grant (2009) and an Education New Zealand postgraduate study abroad award (2009). He joined the research group of Professor Melissa M. Reynolds in 2010 as a post-doctoral research associate and was promoted to Research Scientist – I in 2011. Dr. Damodaran’s research interests include development of biomimetic materials for biomedical applications, tissue engineering nanoscaffolds, controlled drug delivery systems and polymer therapeutics. He has authored over 20 publications in peer reviewed journals and conference proceedings, a book chapter, and one US patent and PCT applications related to polymeric drug delivery system and multifunctional polymers.
Jessica Joslin received her BSc in Chemistry from Adams State College in Alamosa, CO, USA (2005). She is currently a physical chemistry PhD student working for Dr. Reynolds at Colorado State University. She develops spectro scopic and other analytical methods to monitor nitric oxide loading and release processes within solution phase and solid materials. She is interested in probing physical processes associated with functional polymers for biological applications.
Professor Melissa Reynolds is a Boettcher Investigator and Assistant Professor in the Departments of Chemistry and Biomedical Engineering at Colorado State University. She received her PhD in chemistry from the University of Michigan and has over 14 years of experience designing, developing and testing materials for use in medical devices in both industrial and academic settings. She is an inventor on 15 issued/pending patents and has received a Science Award from the National Institutes of Health (USA) in recognition of her work involving the role of nitrite in pathophysiology. Most recently, she was named Educator of the Year by the Colorado Bioscience Association. Her passion, innovation and leadership have led to the formation of multiple start-up companies. Currently, her primary research focus is on the development of therapeutic materials that work synergistically within the body. She is also the CEO and co-founder of Diazamed, an advanced biomaterials company, with the support of CSU Ventures, the commercialisation arm of Colorado State University. She is currently serving as the Biomaterials Editor of SurFACTS in Biomaterials and has authored over 80 publications in peer reviewed journals and conference proceedings.