Unconventional RNA interference – recent approaches to robust RNAi
Posted: 19 October 2011 |
RNA interference (RNAi) is now a standard tool in molecular biology. Short interfering RNAs (siRNAs) for knocking down your favourite human gene are only a couple of mouse-clicks away at your favourite reagent supplier’s website. Moreover, in contrast to initial attempts at siRNA design, these siRNAs usually give potent target gene knockdown. Nevertheless, siRNAs are not always a cure-all; therapeutic settings often require combinatorial treatments and may necessitate effects that are incompatible with standard siRNAs, such as targeted gene up-regulation. Here, we review the features of standard siRNAs before describing three unconventional but therapeutically relevant approaches to RNAi: multi-targeting siRNAs, immunostimulatory siRNAs, and transcription-modulating siRNAs.
Fire and Mello coined the term RNA interference when they discovered that long doublestranded RNAs cause sequence specific gene inhibition in worms1,2. The enzyme Dicer processes such long double-stranded RNAs into short double-stranded ~22 nt duplexes with 2 nt 3’ overhangs – the siRNAs. Argonaute 2 (Ago2) then incorporates one of the siRNA strands and uses the strand as a guide to bind and cleave single-stranded RNAs such as messenger RNAs (mRNAs).


FIGURE 1 siRNA design principles. (A) Highly effective standard siRNAs have a guide strand (in green) with a less thermodynamically stable 5’ than 3’ end (indicated with dashed lines) and position-specific nucleotide preferences (indicated above guide strand). P and OH indicate 5’ phosphate groups and 3’ hydroxyl ends, respectively; blue region indicate seed region. (B) Bi-functional siRNAs targeting a single or (C) two different transcripts. (D) A dual-targeting siRNA where one strand (light green) targets EGFR and the other strand (dark green) targets CCND1
RNA interference (RNAi) is now a standard tool in molecular biology. Short interfering RNAs (siRNAs) for knocking down your favourite human gene are only a couple of mouse-clicks away at your favourite reagent supplier’s website. Moreover, in contrast to initial attempts at siRNA design, these siRNAs usually give potent target gene knockdown. Nevertheless, siRNAs are not always a cure-all; therapeutic settings often require combinatorial treatments and may necessitate effects that are incompatible with standard siRNAs, such as targeted gene up-regulation. Here, we review the features of standard siRNAs before describing three unconventional but therapeutically relevant approaches to RNAi: multi-targeting siRNAs, immunostimulatory siRNAs, and transcription-modulating siRNAs.
Fire and Mello coined the term RNA interference when they discovered that long doublestranded RNAs cause sequence specific gene inhibition in worms1,2. The enzyme Dicer processes such long double-stranded RNAs into short double-stranded ~22 nt duplexes with 2 nt 3’ overhangs – the siRNAs. Argonaute 2 (Ago2) then incorporates one of the siRNA strands and uses the strand as a guide to bind and cleave single-stranded RNAs such as messenger RNAs (mRNAs). Thus, RNA-loaded Ago2 forms the main component of the so-called RNA-induced silencing complex (RISC)3. Importantly, Ago2 requires near-perfect complementarity between its siRNA guide strand and RNA target, as single mismatches – particularly at the cleavage site in the middle of the guide strand – can prevent cleavage4.
In humans, long double-stranded RNAs induce a potent innate immune response and are therefore impracticable RNAi triggers5. Synthetic siRNAs can bypass this response6, but in contrast to long double-stranded RNAs in worms, not all synthetic siRNAs effectively down-regulate their targets7. Although puzzling at the time, studies have identified at least three factors that are important for siRNA function.
First, Ago2 (Eukaryotic translation initiation factor 2C, 2 (EIF2C2) in humans) must incorporate the correct strand from the siRNA duplex; that is, the ‘guide’ strand with antisense complementarity to the siRNA’s intended target. Instead of randomly incorporating strands, Ago2 favours the duplex strand whose 5’ end is least stable8,9, but Ago2 does not itself select this strand. In flies, an auxiliary protein called R2D2 identifies and binds the duplex end that is most stable while the fly-specific Dicer-homologue Dicer 2 binds the other end10. Together, Dicer 2 and R2D2 orient and hand the duplex to Ago2. Humans lack a clear R2D2 homologue; instead, human Dicer1 itself seems to act as the stability sensor11,12. Specifically, and in contrast to flies, Dicer1 binds the least stable duplex end while an additional factor (TARBP2/TRBP or PRKRA/PACT) binds the other end before hand-off to Ago212. After hand-off, both human and fly Ago2 use their catalytic ‘slicer’ activity to cleave the other ‘passenger’ strand13-15, which then dissociates and, in flies, is degraded by a protein complex named C3PO (naturally)16.
Second, the duplex should preferably contain certain nucleotides at certain positions. Although these positional nucleotide preferences are partially related to the first criterion of differential duplex end stability – effective siRNAs tend to have, respectively, A or U and C or G at the 5’ and 3’ ends of their intended guide strand – statistical analyses have also identified positional preferences well within the duplex (Figure 1A)17,18. Few of these nucleotide patterns within the duplex currently have plausible biological explanations; one exception is the preference for Uracil next to the Ago2 cleavage site, which likely relates to endonuclease cleavage preferences17. These position-specific nucleotide patterns are nevertheless the most important features for identifying effective siRNAs19.
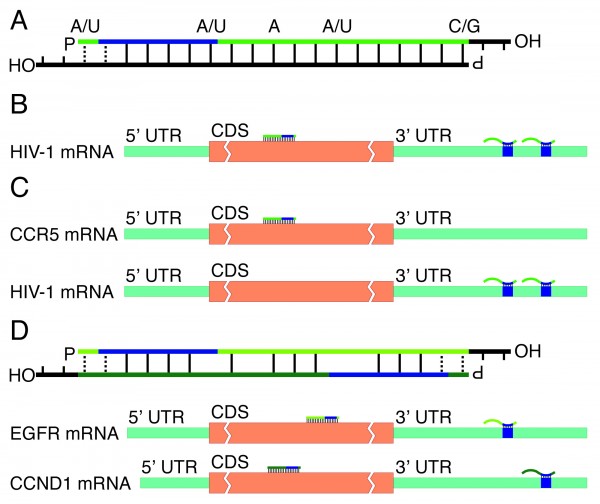
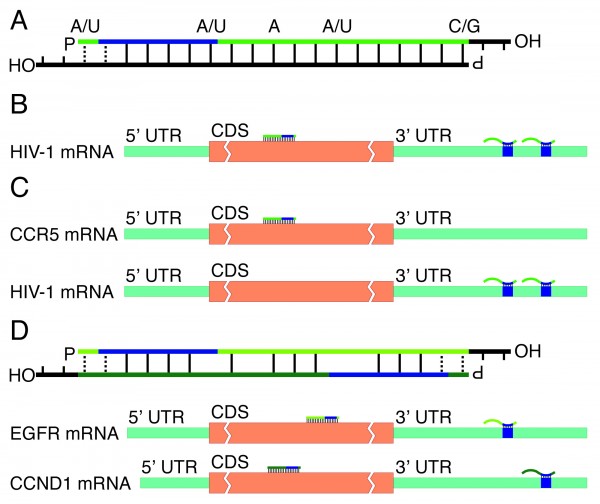
FIGURE 1 siRNA design principles. (A) Highly effective standard siRNAs have a guide strand (in green) with a less thermodynamically stable 5’ than 3’ end (indicated with dashed lines) and position-specific nucleotide preferences (indicated above guide strand). P and OH indicate 5’ phosphate groups and 3’ hydroxyl ends, respectively; blue region indicate seed region. (B) Bi-functional siRNAs targeting a single or (C) two different transcripts. (D) A dual-targeting siRNA where one strand (light green) targets EGFR and the other strand (dark green) targets CCND1
Third, as single stranded RNAs such as mRNAs can base-pair with themselves and form stable RNA structures, the duplex should target a region that is accessible for binding20-23. Similarly, guide-strands that form stable selfinteractions tend to be less effective than guide-strands that remain unstructured17,21,24,25. Studies have also observed that the duplex itself should not be too stable and preferably contain 30 – 50 per cent GCs17,24. This result suggests that the guide strand should be free to easily dissociate from its passenger strand and intended target site. However, the result may also simply reflect that the target site should be accessible, as GC-rich regions tend to form more stable RNA structures than GC-poor regions do. Supporting this latter conclusion, siRNAs chemically modified to form more stable duplexes have increased knockdown efficacy compared with identical but unmodified siRNAs26.
A functional siRNA should meet all these criteria. Consequently, current siRNA designs are based on machine learning algorithms such as artificial neural networks or support vector machines trained to recognise the features of highly effective siRNAs18,19,24,27,28. Synthetic siRNAs are also typically chemically modified; for example, to effect correct strand inclusion29. Together, chemical modifications and machine learning-based design have made synthetic siRNAs into the current off-the-shelf solution for gene knockdown.
Multi-targeting siRNAs
In therapeutic settings, combinatorial treat – ments are often useful or necessary. Treating cancer may for example require knocking down multiple oncogenes, whereas viruses, such as HIV-1, can escape single siRNA-mediated knockdown by accumulating mutations at the siRNA target site30,31.
Pools of different siRNAs are one popular approach to combinatorial RNAi, but such pools can amplify one of siRNAs’ downsides – offtarget effects. Once incorporated into RISC, siRNA guide strands are indistinguishable from endogenous microRNAs (miRNAs) and siRNAs therefore share miRNAs’ regulatory properties32. As a single animal miRNA often regulates hundreds of targets, a single siRNA can have, and typically has, hundreds of off-targets33-35. Such off-targets can create false positives in phenotypic screens and undesired side effects in therapeutic settings and are therefore usually unwanted36,37. If controlled, however, this miRNAlike regulation can add additional functions to a single siRNA molecule; for example, the ability to target more than one gene38.
Controlling siRNA off-target effects requires understanding the principles behind miRNA targeting. MicroRNAs primarily target short 6-7 nt ‘seed’ sites with perfect complementarity to the miRNAs’ 5’ end, located in mRNA 3’ untranslated regions (UTRs)32. Not all such seed sites are functional, however; factors that increase miRNAs’ regulatory effect are additional base pairing between miRNA and mRNA next to the seed or in the miRNA 3’ region, a high local AU content surrounding the seed site, additional seed sites, and a short 13-35 nts distance between such additional seed sites32,39,40. Using these principles, Ehsani and colleagues designed a bi-functional siRNA that simultaneously targeted multiple miRNA-like seed sites and a perfectly matched cleavage site within HIV-1 (Figure 1B-C). This and a similar siRNA that targeted both HIV-1 and HIV’s co-receptor CCR5 could both robustly inhibit HIV-replication41.
An alternative approach to dual-targeting siRNAs is to create duplexes where both strands target different genes (Figure 1D). Both a siRNA’s strands can potentially enter RISC and for regular siRNAs, passenger strand entry can be a major source of off-target effects34. By identifying two target sites that have near-perfect complementarity, however, one can create a duplex with two guide strands against two separate targets. Building on a proof-of-concept study by Hossbach and colleagues42, Tiemann and colleagues developed a design algorithm that reliably creates such dual-targeting siRNAs43. These dual-targeting siRNAs were as effective as similar single-targeting siRNAs. Moreover, compared with pools of singletargeting siRNAs, dual-targeting siRNAs had reduced off-target effects. Comparable duplex end stability was a key feature of the dualtargeting siRNAs, but ensuring that both strands shared the positional nucleotide preferences of effective standard siRNAs and that the strands had seed sites within their intended targets’ 3’ UTRs also improved knockdown efficacy. Computational analyses showed that 55-87 per cent of all gene pairs could be targeted by such dual-targeting siRNAs, depending on duplex complementarity. As Ehsani and colleagues’ bi-functional siRNAs also can target about 86 per cent of all gene pairs41, dual-targeting siRNAs can be an alternative to siRNA pools for most combinatorial RNAi therapeutics.
Immunostimulatory siRNAs
Immunostimulation by siRNAs was initially discovered as an off-target effect of experimental RNAi44 and is a matter of concern in RNAi-based therapeutics. Although the immuno stimulatory effect is often unwanted, combining the effects of gene specific silencing (RNAi) and immunostimulation by siRNAs can be beneficial and offers a new therapeutic strategy. RNA species can be recognised by several different molecular sensors of the innate immune system, including PKR, RIG-1, MDA-5, and toll receptors (TLRs) 3, 7, and 8 (reviewed in45-48). PKR, RIG-1, and MDA-5 are expressed in the cytosol of non-immune cells and recognise longer dsRNAs (>30 bp) without sequencespecificity48. TLRs 7 and 8 are expressed in endosomes of immune cells, recognise single stranded RNAs (ssRNAs), and play dominating roles in mediating an immune response to conventional ~21 nt siRNAs as well as longer Dicer-substrate siRNAs49-52.
TLR7/8-mediated immunostimulation by siRNAs show sequence- and structuredependency and furthermore depend on cell type and mode of delivery53. The mechanism is thought to be that double stranded siRNAs delivered by lipid-based vehicles are internalised in endosomes where a fraction is degraded to ssRNAs49 (Figure 2). Depending on the sequence, the ssRNAs can then be recognised by TLR7/8 and initiate a signalling cascade leading to induction of interferons (IFNα and β) or inflammatory cytokines (e.g. TNF-α and IL-12) and ultimately to activation of immune cells49. Another fraction of siRNAs apparently stays intact, escapes the endosome, and exert specific target knockdown through RNAi. Several groups have searched for immunostimulatory motifs in siRNAs49,50,52,54-56. Although no consistent motif has been defined, there is a general agreement on the stimulatory role of GU- or U-rich sequences (Table 1).
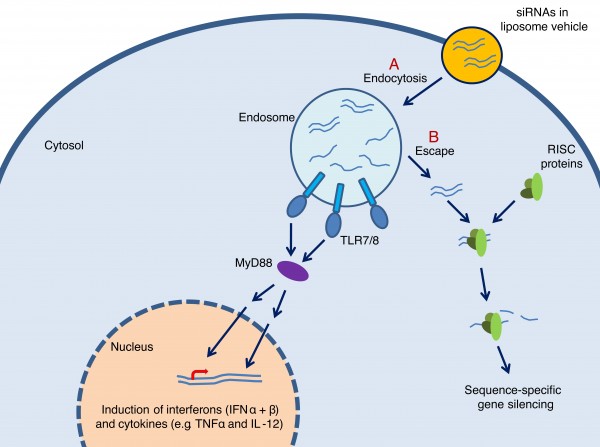
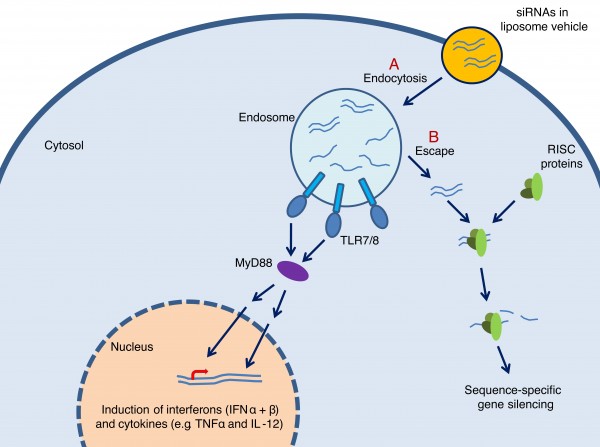
FIGURE 2 Mechanism for TLR7/8-mediated immunostimulation by siRNAs. (A) siRNAs delivered by liposome vehicles are internalised by endocytosis. Inside the endosome, some siRNAs are degraded to single stranded RNAs that can be recognised by the internal domains of TLR7/8 leading to activation of the receptors. Upon activation, TLR7/8 initiate a signalling cascade involving the adaptor molecule MyD88 in addition to other signalling molecules and transcription factors. This signalling cascade ultimately leads to transcription of interferons (IFNα/β) and cytokines (e.g. TNFα and IL-12) thereby inducing antiviral and antitumoral responses. (B) Some siRNAs escape the endosome, are recognised by RISC-proteins in the cytosol, and silence target genes by RNAi
Gantier et al52 have pointed out that any siRNA that is not already highly immuno – stimulatory can be made highly immuno stimulatory by adding a miRNA-like U-rich bulge to the sense strand. Importantly, they further showed that Dicer-substrate siRNAs containing the U-rich bulge were just as efficient at knocking down their target genes, meaning that this modification was compatible with RNAi.
Table 1. TLR7 and/or TLR8 immunostimulatory motifs in siRNAs | |||
Immunostimulatory motif (5´-3´) | Comment | Reference | |
GUCCUUCAA | 9 bases at the 3´ end of the sense strand of 21 nt siRNA | Hornung V et al (2005) | |
UGUGU | Sense strand of 19 nt siRNA | Judge AD et al (2005) | |
UGUUUUAAU(/CUU)XUUGUUAAAA(/UUUU)UU | 21 nt siRNA, 2 base 3´overhangs | Goodchild A et al (2009) | |
GU-rich 4-mers | 18 nt siRNA, activate TLR7/8 | Forsbach A et al (2008) | |
AU-rich 4-mers | 18 nt siRNA, activate TLR8 | Forsbach A et al (2008) | |
Internal miRNA-like U-bulge | Asymmetric Dicer-substrate siRNA | Gantier MP et al (2010) | |
UXUCU | Asymmetric Dicer-substrate siRNA | Khairuddin N et al (2011) |
Therapeutic potential of immunostimulatory siRNAs
The fact that TLR7/8-stimulation leads to induction of multiple anti-viral genes and activation of several different immune cells implies that this effect alone has therapeutic potential in the treatment of viral-infections and cancer. Indeed, small synthetic drugs that are recognised by TLR7/8 are already in clinical use for treating genital warts caused by human papillomavirus (HPV) and for treating malignant skin cancer (reviewed in57). It seems likely that bifunctional siRNAs could provide attractive alternative drugs in combining the effect of a TLR7/8-mediated immune response with specific gene silencing. Khairuddin et al51 used this strategy to target HPV-induced cervical cancer. They tested 15 Dicer-substrate siRNAs that targeted essential viral genes and selected one candidate bi-functional siRNA that efficiently knocked down the target gene and also potently induced the TLR7/8 immune response in human and murine cancer cell-lines. Using a mouse-model they further showed that systemic delivery of the bi-functional siRNA led to reduced tumour growth in established tumours and also prohibited tumour formation in healthy individuals, clearly demonstrating an antitumoral effect. Interestingly, this effect was prolonged when treating with the bi-functional siRNA compared to treating with a nontargeting highly immunostimulatory siRNA, thus supporting the idea that bi-functional siRNAs can enhance cancer treatment.
Recently, Stewart et al58 successfully used the same strategy to target avian influenza H5N1 in chicken cells. They found the immuno – stimulatory effect of the H5N1-targeted siRNA to be dependent on TLR3 rather than TLR7/8 in chicken cells, indicating that TLR-functions differ between species. They further point to the possibility of designing immunostimulatory siRNAs that target conserved regions in the influenza A genome, thereby making it possible to target several strains across different species; e.g. both the H5N1 and the H1N1 influenza strains in human as well as poultry. This could have great therapeutic potential in cases where viral infections are transmitted between species.
Transcription-modulating siRNAs
While the human genome project revealed that less than two per cent of the human genome consists of protein-coding genes, more than 90 per cent of the genome can be transcribed and large parts of the genome give rise to non-coding RNAs (ncRNAs) with diverse functions (reviewed in59,60). Transcription occurs in both directions and overlapping transcripts yielding sense/antisense transcripts from the same genomic location are common61-63. Long ncRNAs can regulate gene expression by recruiting chromatinmodifying complexes to target genes or genomic loci, thereby inducing epigenetic silencing64-67. In some cases, long ncRNAs are transcribed from intergenic regions (large intergenic ncRNAs, or lincRNAs) and induce epigenetic silencing of target genomic loci in trans64. In other cases long ncRNAs are promoter-associated and regulate transcription of nearby genes in cis63,65.
While several groups have reported that small dsRNAs like siRNAs, shRNAs, and miRNAs can induce transcriptional silencing67-72 or gene activation73-77 in a sequence-specific manner, some groups have pointed out that this form of transcriptional regulation is dependent on ncRNAs65-67,71,78,79. Thus, the mechanism seems to be that small dsRNAs that are complementary to promoter regions in fact target ncRNAs that are either sense66,67,71 or antisense65,78,79 to the target gene. The ncRNAs then recruit chromatinmodifying complexes that either silence or activate transcription of the target gene. Apparently, this form of regulation is long-lasting and can be passed on to daughter cells63,67,74, a feature which makes long ncRNAs promising therapeutic targets in human diseases.
Interestingly, miRNAs have predicted target sites within gene promoters indicating that they could be involved in epigenetic regulation of gene transcription68,70,80. Furthermore, it has been proposed that the balance between sense and antisense transcripts may decide whether a gene is activated or epigenetically silenced65. Perhaps deregulated miRNAs, associated with human diseases like cancer, could be disturbing this balance leading to long-term silencing of tumour-suppressor genes or activation of oncogenes, thereby contributing to cancer development.
Therapeutic potential of small dsRNAs that induce epigenetic regulation of target genes
As a large population of ncRNAs in human cells i) is implicated in long-term gene regulation, ii) may be involved in deregulation of diseaserelated genes, and iii) can be targeted by small dsRNAs in a sequence specific manner, these ncRNAs can be attractive therapeutic targets. One strategy is to target ncRNAs to activate genes that are epigenetically silenced in human diseases. Indeed, this has already been tried and some examples are activation of E-cadherin and p21 – genes which are often epigenetically silenced in human cancers73,74,76,77. Activation of E-cadherin in human cancer cell lines by small dsRNAs led to reduced invasion and migration in bladder and prostate cancer cells76,77 as well as induced apoptosis and reduced cell growth in breast cancer cells73.
Another strategy is to silence diseaserelated genes like oncogenes or genes that are necessary for viral replication. Promotertargeting siRNAs against c-myc, an oncogene which is often up-regulated in human cancers, gave growth arrest, loss of clonogenic potential, and senescence in human prostate cancer cells71. Also, Yamagishi et al69 used a shRNA to silence HIV-1 expression in a T-cell line and observed long-term silencing lasting for at least one year, thus offering an alternative therapeutic strategy for preventing HIV-progression.
The role of ncRNAs in epigenetic regulation represents a promising new era for RNA-based therapies even though there are clearly many challenges to overcome.
One of the challenges is finding rules for designing activating or silencing small dsRNAs. It has been proposed that siRNAs that target the antisense strand of the promoter induce gene activation whereas siRNAs that target the promoter sense strand induce silencing65. It has also been suggested that siRNA-mediated activation or silencing depend on the initial expression levels of the target gene81. Recognising the complexity of gene regulation, it seems inevitable that the features which make siRNAs either activating or silencing will depend on the context.
Concluding remarks
Clearly, we are only beginning to understand the complexity of gene regulation and it seems likely that endogenous small dsRNAs play widespread roles in regulation through their ability to recognise different RNA-species based on sequence complementarity. As outlined above, improved insight into RNA’s regulatory roles in general and dsRNAs’ specific pathways and functions have suggested several novel approaches to robust RNA-based therapeutics. The major hurdle for taking RNAi to the clinic therefore still appears to be delivery.
References
1. Fire A, Xu S, Montgomery MK, Kostas SA, Driver SE, Mello CC. Potent and specific genetic interference by doublestranded RNA in Caenorhabditis elegans. Nature. 1998 Feb 19;391(6669):806-811
2. Czech B, Hannon GJ. Small RNA sorting: matchmaking for Argonautes. Nat. Rev. Genet. 2011 Jan;12(1):19-31
3. Rivas FV, Tolia NH, Song J-J, Aragon JP, Liu J, Hannon GJ, et al. Purified Argonaute2 and an siRNA form recombinant human RISC. Nat. Struct. Mol. Biol. 2005 Apr;12(4):340-349
4. Haley B, Zamore PD. Kinetic analysis of the RNAi enzyme complex. Nat. Struct. Mol. Biol. 2004 Jul;11(7):599-606
5. Gantier MP, Williams BRG. The response of mammalian cells to double-stranded RNA. Cytokine Growth Factor Rev. 2007 Dec;18(5-6):363-371
6. Elbashir SM, Harborth J, Lendeckel W, Yalcin A, Weber K, Tuschl T. Duplexes of 21-nucleotide RNAs mediate RNA interference in cultured mammalian cells. Nature. 2001 May 24;411(6836):494-498
7. Holen T, Amarzguioui M, Wiiger MT, Babaie E, Prydz H. Positional effects of short interfering RNAs targeting the human coagulation trigger Tissue Factor. Nucleic Acids Res. 2002 Apr 15;30(8):1757-1766
8. Khvorova A, Reynolds A, Jayasena SD. Functional siRNAs and miRNAs exhibit strand bias. Cell. 2003 Oct 17;115(2):209-216
9. Schwarz DS, Hutvágner G, Du T, Xu Z, Aronin N, Zamore PD. Asymmetry in the assembly of the RNAi enzyme complex. Cell. 2003 Oct 17;115(2):199-208
10. Tomari Y, Matranga C, Haley B, Martinez N, Zamore PD. A protein sensor for siRNA asymmetry. Science. 2004 Nov 19;306(5700):1377-1380
11. Sakurai K, Amarzguioui M, Kim D-H, Alluin J, Heale B, Song M-sun, et al. A role for human Dicer in pre-RISC loading of siRNAs. Nucleic Acids Res. 2011 Mar;39(4):1510-1525
12. Noland CL, Ma E, Doudna JA. siRNA Repositioning for Guide Strand Selection by Human Dicer Complexes. Mol. Cell. 2011 Jul 8;43(1):110-121.
13. Miyoshi K, Tsukumo H, Nagami T, Siomi H, Siomi MC. Slicer function of Drosophila Argonautes and its involvement in RISC formation. Genes Dev. 2005 Dec 1;19(23):2837-2848
14. Rand TA, Petersen S, Du F, Wang X. Argonaute2 cleaves the anti-guide strand of siRNA during RISC activation. Cell. 2005 Nov 18;123(4):621-629
15. Matranga C, Tomari Y, Shin C, Bartel DP, Zamore PD. Passenger-strand cleavage facilitates assembly of siRNA into Ago2-containing RNAi enzyme complexes. Cell. 2005 Nov 18;123(4):607-620
16. Liu Y, Ye X, Jiang F, Liang C, Chen D, Peng J, et al. C3PO, an endoribonuclease that promotes RNAi by facilitating RISC activation. Science. 2009 Aug 7;325(5941):750-753
17. Reynolds A, Leake D, Boese Q, Scaringe S, Marshall WS, Khvorova A. Rational siRNA design for RNA interference. Nat. Biotechnol. 2004 Mar;22(3):326-330
18. Saetrom P, Snøve O Jr. A comparison of siRNA efficacy predictors. Biochem. Biophys. Res. Commun. 2004 Aug 13;321(1):247-253
19. Peek AS. Improving model predictions for RNA interference activities that use support vector machine regression by combining and filtering features. BMC Bioinformatics. 2007;8:182
20. Heale BSE, Soifer HS, Bowers C, Rossi JJ. siRNA target site secondary structure predictions using local stable substructures. Nucleic Acids Res. 2005;33(3):e30
21. Tafer H, Ameres SL, Obernosterer G, Gebeshuber CA, Schroeder R, Martinez J, et al. The impact of target site accessibility on the design of effective siRNAs. Nat. Biotechnol. 2008 May;26(5):578-583
22. Shao Y, Chan CY, Maliyekkel A, Lawrence CE, Roninson IB, Ding Y. Effect of target secondary structure on RNAi efficiency. RNA. 2007 Oct;13(10):1631-1640
23. Ameres SL, Martinez J, Schroeder R. Molecular basis for target RNA recognition and cleavage by human RISC. Cell. 2007 Jul 13;130(1):101-112.
24. Saetrom P. Predicting the efficacy of short oligonucleotides in antisense and RNAi experiments with boosted genetic programming. Bioinformatics. 2004 Nov 22;20(17):3055-3063
25. Patzel V, Rutz S, Dietrich I, Köberle C, Scheffold A, Kaufmann SHE. Design of siRNAs producing unstructured guide-RNAs results in improved RNA interference efficiency. Nat. Biotechnol. 2005 Nov;23(11):1440-1444
26. Petri S, Dueck A, Lehmann G, Putz N, Rüdel S, Kremmer E, et al. Increased siRNA duplex stability correlates with reduced off-target and elevated on-target effects. RNA. 2011 Apr;17(4):737-749
27. Huesken D, Lange J, Mickanin C, Weiler J, Asselbergs F, Warner J, et al. Design of a genome-wide siRNA library using an artificial neural network. Nat. Biotechnol. 2005 Aug;23(8):995-1001
28. McQuisten KA, Peek AS. Comparing artificial neural networks, general linear models and support vector machines in building predictive models for small interfering RNAs. PLoS ONE. 2009;4(10):e7522
29. Watts JK, Deleavey GF, Damha MJ. Chemically modified siRNA: tools and applications. Drug Discov. Today. 2008 Oct;13(19-20):842-855
30. Boden D, Pusch O, Lee F, Tucker L, Ramratnam B. Human immunodeficiency virus type 1 escape from RNA interference. J. Virol. 2003 Nov;77(21):11531-11535
31. Song E, Lee S-K, Dykxhoorn DM, Novina C, Zhang D, Crawford K, et al. Sustained small interfering RNAmediated human immunodeficiency virus type 1 inhibition in primary macrophages. J. Virol. 2003 Jul;77(13):7174-7181.
32. Bartel DP. MicroRNAs: target recognition and regulatory functions. Cell. 2009 Jan 23;136(2):215-233
33. Birmingham A, Anderson EM, Reynolds A, Ilsley-Tyree D, Leake D, Fedorov Y, et al. 3’ UTR seed matches, but not overall identity, are associated with RNAi off-targets. Nat. Methods. 2006 Mar;3(3):199-204
34. Jackson AL, Bartz SR, Schelter J, Kobayashi SV, Burchard J, Mao M, et al. Expression profiling reveals off-target gene regulation by RNAi. Nat. Biotechnol. 2003 Jun;21(6):635-637
35. Jackson AL, Burchard J, Schelter J, Chau BN, Cleary M, Lim L, et al. Widespread siRNA “off-target” transcript silencing mediated by seed region sequence complementarity. RNA. 2006 Jul;12(7):1179-1187
36. Fedorov Y, Anderson EM, Birmingham A, Reynolds A, Karpilow J, Robinson K, et al. Off-target effects by siRNA can induce toxic phenotype. RNA. 2006 Jul;12(7):1188- 1196
37. Lin X, Ruan X, Anderson MG, McDowell JA, Kroeger PE, Fesik SW, et al. siRNA-mediated off-target gene silencing triggered by a 7 nt complementation. Nucleic Acids Res. 2005;33(14):4527-4535
38. Passioura T, Gozar MM, Goodchild A, King A, Arndt GM, Poidinger M, et al. Interfering ribonucleic acids that suppress expression of multiple unrelated genes. BMC Biotechnol. 2009;9:57
39. Grimson A, Farh KK-H, Johnston WK, Garrett-Engele P, Lim LP, Bartel DP. MicroRNA targeting specificity in mammals: determinants beyond seed pairing. Mol. Cell. 2007 Jul 6;27(1):91-105
40. Saetrom P, Heale BSE, Snøve O Jr, Aagaard L, Alluin J, Rossi JJ. Distance constraints between microRNA target sites dictate efficacy and cooperativity. Nucleic Acids Res. 2007;35(7):2333-2342
41. Ehsani A, Saetrom P, Zhang J, Alluin J, Li H, Snøve O Jr, et al. Rational design of micro-RNA-like bifunctional siRNAs targeting HIV and the HIV coreceptor CCR5. Mol. Ther. 2010 Apr;18(4):796-802
42. Hossbach M, Gruber J, Osborn M, Weber K, Tuschl T. Gene silencing with siRNA duplexes composed of target-mRNA-complementary and partially palindromic or partially complementary singlestranded siRNAs. RNA Biol. 2006 Apr;3(2):82-89
43. Tiemann K, Höhn B, Ehsani A, Forman SJ, Rossi JJ, Saetrom P. Dual-targeting siRNAs. RNA. 2010 Jun;16(6):1275-1284
44. Sledz CA, Holko M, de Veer MJ, Silverman RH, Williams BRG. Activation of the interferon system by shortinterfering RNAs. Nat Cell Biol. 2003;5(9):834-839
45. Gantier MP, Williams BRG. The response of mammalian cells to double-stranded RNA. Cytokine Growth Factor Rev. 2007;18(5-6):363-371
46. Sioud M. RNA interference and innate immunity. Advanced Drug Delivery Reviews. 2007 Mar 30;59(2-3):153-163
47. Marques JT, Williams BRG. Activation of the mammalian immune system by siRNAs. Nat Biotech. 2005 Nov;23(11):1399-1405
48. Schlee M, Hornung V, Hartmann G. siRNA and isRNA: Two Edges of One Sword. Mol Ther. 2006 Oct;14(4):463-470
49. Sioud M. Induction of Inflammatory Cytokines and Interferon Responses by Double-stranded and Singlestranded siRNAs is Sequence-dependent and Requires Endosomal Localization. Journal of Molecular Biology. 2005 May 20;348(5):1079-1090
50. Hornung V, Guenthner-Biller M, Bourquin C, Ablasser A, Schlee M, Uematsu S, et al. Sequence-specific potent induction of IFN-[alpha] by short interfering RNA in plasmacytoid dendritic cells through TLR7. Nat Med. 2005 Mar;11(3):263-270
51. Khairuddin N, Gantier MP, Blake SJ, Wu SY, Behlke MA, Williams BR, et al. siRNA-induced immunostimulation through TLR7 promotes antitumoral activity against HPV-driven tumors in vivo. Immunol Cell Biol [Internet]. 2011 Mar 22 [cited 2011 Jun 1];Available from: http://dx.doi.org/10.1038/icb.2011.19
52. Gantier MP, Tong S, Behlke MA, Irving AT, Lappas M, Nilsson UW, et al. Rational Design of Immuno – stimulatory siRNAs. Mol Ther. 2010 Apr;18(4):785-795
53. Nguyen DN, Chen SC-Y, Lu J, Goldberg M, Kim P, Sprague A, et al. Drug Delivery–mediated Control of RNA Immunostimulation. Mol Ther. 2009 Sep; 17(9):1555-1562
54. Forsbach A, Nemorin J-G, Montino C, Müller C, Samulowitz U, Vicari AP, et al. Identification of RNA Sequence Motifs Stimulating Sequence-Specific TLR8- Dependent Immune Responses. The Journal of Immunology. 2008 Mar 15;180(6):3729 -3738
55. Judge AD, Sood V, Shaw JR, Fang D, McClintock K, MacLachlan I. Sequence-dependent stimulation of the mammalian innate immune response by synthetic siRNA. Nat Biotech. 2005 Apr;23(4):457-462
56. Goodchild A, Nopper N, King A, Doan T, Tanudji M, Arndt GM, et al. Sequence determinants of innate immune activation by short interfering RNAs. BMC Immunol. 10:40-40
57. Smits ELJM, Ponsaerts P, Berneman ZN, Van Tendeloo VFI. The Use of TLR7 and TLR8 Ligands for the Enhancement of Cancer Immunotherapy. The Oncologist. 2008;13(8):859 -875
58. Stewart CR, Karpala AJ, Lowther S, Lowenthal JW, Bean AG. Immunostimulatory Motifs Enhance Antiviral siRNAs Targeting Highly Pathogenic Avian Influenza H5N1. PLoS One. 2011 Jul 1;6(7)
59. Wilusz JE, Sunwoo H, Spector DL. Long noncoding RNAs: functional surprises from the RNA world. Genes Dev. 2009 Jul 1;23(13):1494-1504
60. Kapranov P, Willingham AT, Gingeras TR. Genome-wide transcription and the implications for genomic organization. Nat. Rev. Genet. 2007 Jun;8(6):413-423.
61. Kapranov P, Cheng J, Dike S, Nix DA, Duttagupta R, Willingham AT, et al. RNA Maps Reveal New RNA Classes and a Possible Function for Pervasive Transcription. Science. 2007 Jun 8;316(5830):1484 -1488
62. RIKEN Genome Exploration Research Group and Genome Science Group (Genome Network Project Core Group) and the FANTOM Consortium, Katayama S, Tomaru Y, Kasukawa T, Waki K, Nakanishi M, et al. Antisense Transcription in the Mammalian Transcriptome. Science. 2005;309(5740):1564 -1566
63. Yu W, Gius D, Onyango P, Muldoon-Jacobs K, Karp J, Feinberg AP, et al. Epigenetic silencing of tumour suppressor gene p15 by its antisense RNA. Nature. 2008 Jan 10;451(7175):202-206
64. Khalil AM, Guttman M, Huarte M, Garber M, Raj A, Rivea Morales D, et al. Many human large intergenic noncoding RNAs associate with chromatin-modifying complexes and affect gene expression. Proc Natl Acad Sci U S A. 2009 Jul 14;106(28):11667-11672
65. Morris KV, Santoso S, Turner A-M, Pastori C, Hawkins PG. Bidirectional Transcription Directs Both Transcriptional Gene Activation and Suppression in Human Cells. PLoS Genet. 2008 Nov;4(11)
66. Han J, Kim D, Morris KV. Promoter-associated RNA is required for RNA-directed transcriptional gene silencing in human cells. Proc Natl Acad Sci U S A. 2007 Jul 24;104(30):12422-12427
67. Hawkins PG, Santoso S, Adams C, Anest V, Morris KV. Promoter targeted small RNAs induce long-term transcriptional gene silencing in human cells. Nucleic Acids Res. 2009 May;37(9):2984-2995
68. Kim DH, Sætrom P, Snøve O, Rossi JJ. MicroRNA-directed transcriptional gene silencing in mammalian cells. Proc Natl Acad Sci U S A. 2008 Oct 21;105(42):16230-16235
69. Yamagishi M, Ishida T, Miyake A, Cooper DA, Kelleher AD, Suzuki K, et al. Retroviral delivery of promoter-targeted shRNA induces long-term silencing of HIV-1 transcription. Microbes and Infection. 2009 Apr;11(4):500-508
70. Younger ST, Corey DR. Transcriptional gene silencing in mammalian cells by miRNA mimics that target gene promoters. Nucleic Acids Research [Internet]. 2011 Mar 22 [cited 2011 Jun 16];Available from: http://nar.oxfordjournals.org/content/early/2011/03/22 /nar.gkr155.abstract
71. Napoli S, Pastori C, Magistri M, Carbone GM, Catapano CV. Promoter-specific transcriptional interference and cmyc gene silencing by siRNAs in human cells. EMBO J. 2009 Jun 17;28(12):1708-1719
72. Morris KV, Chan SW-L, Jacobsen SE, Looney DJ. Small Interfering RNA-Induced Transcriptional Gene Silencing in Human Cells. Science. 2004;305(5688):1289 -1292
73. Junxia W, Ping G, Yuan H, Lijun Z, Jihong R, Fang L, et al. Double strand RNAıguided endogeneous Eıcadherin upıregulation induces the apoptosis and inhibits proliferation of breast carcinoma cells in vitro and in vivo. Cancer Science. 2010 Aug 1;101(8):1790-1796
74. Li L-C, Okino ST, Zhao H, Pookot D, Place RF, Urakami S, et al. Small dsRNAs induce transcriptional activation in human cells. Proc Natl Acad Sci U S A. 2006 Nov 14;103(46):17337-17342
75. Place RF, Li L-C, Pookot D, Noonan EJ, Dahiya R. MicroRNA-373 induces expression of genes with complementary promoter sequences. Proc Natl Acad Sci USA. 2008 Feb 5;105(5):1608-1613
76. Mao Q, Zheng X, Yang K, Qin J, Bai Y, Jia X, et al. Suppression of Migration and Invasion of PC3 Prostate Cancer Cell Line via Activating E-Cadherin Expression by Small Activating RNA. Cancer Invest. 2010 Nov;28(10):1013-1018
77. Mao Q, Li Y, Zheng X, Yang K, Shen H, Qin J, et al. Upregulation of E-cadherin by small activating RNA inhibits cell invasion and migration in 5637 human bladder cancer cells. Biochemical and Biophysical Research Communications. 2008 Oct 31;375(4):566-570
78. Chu Y, Yue X, Younger ST, Janowski BA, Corey DR. Involvement of argonaute proteins in gene silencing and activation by RNAs complementary to a non-coding transcript at the progesterone receptor promoter. Nucleic Acids Res. 2010 Nov;38(21):7736-7748
79. Schwartz JC, Younger ST, Nguyen N-B, Hardy DB, Monia BP, Corey DR, et al. Antisense transcripts are targets for activating small RNAs. Nat Struct Mol Biol. 2008 Aug;15(8):842-848
80. Younger ST, Pertsemlidis A, Corey DR. Predicting potential miRNA target sites within gene promoters. Bioorg Med Chem Lett. 2009 Jul 15;19(14):3791-3794
81. Janowski BA, Younger ST, Hardy DB, Ram R, Huffman KE, Corey DR. Activating gene expression in mammalian cells with promoter-targeted duplex RNAs. Nat Chem Biol. 2007 Mar;3(3):166-173
About the Authors
Marie Lundbæk received her MSc in molecular biology from NTNU in 2006. She is currently a PhD student in Pål Sætrom’s group at NTNU, where she works on microRNAs’ roles in cell cycle regulation.
Professor Pål Sætrom has a MSc in computer science (2001) and a DPhil in bioinformatics (2005) from the Norwegian University of Science and Technology (NTNU). Pål then joined Professor John J. Rossi’s lab at the City of Hope, where he worked on algorithms for predicting microRNA genes and target sites. Returning to Norway 2007, he joined NTNU’s Department of Cancer Research on career fellowships from NTNU and the Norwegian Research Council. His group has since combined computational analyses with experimental work to study small RNAs and their regulatory roles.