Flow cytometry for the optimisation of microbial recombinant protein production
Posted: 28 October 2014 | | No comments yet
Flow cytometry is an extremely useful technique for the rapid analysis of many cell types. Here, the applications of this technique for the monitoring and optimisation of microbial recombinant protein production processes are discussed.
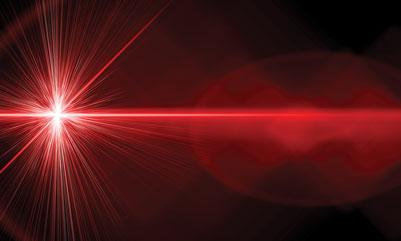
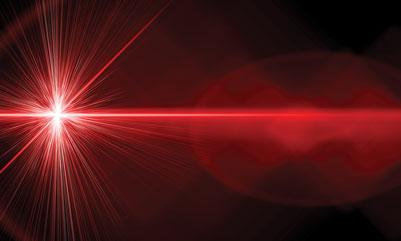
Recombinant protein production in microbial hosts is an essential process in the biopharmaceutical sector, and has been since the Food and Drug Administration (FDA) approval of recombinant human insulin in 1982. Recombination of a gene encoding a therapeutic protein of interest into a microbial host (most typically the bacterium Escherichia coli or the yeast Saccharomyces cerevisiae, although host range is constantly expanding) permits the production of that protein in a process more simple and frequently at lower cost than if a mammalian cell host (such as the Chinese Hamster Ovary cell) was required. Microbial production of therapeutic proteins is currently used to manufacture around 30% of all protein biopharmaceutical products, and is still the desired route for recombinant proteins that do not require extensive glycosylation or other post-translational modifications for correct function1,2.
Flow cytometry is a technique for determining optical and fluorescent properties of particles, usually biological particles such as cells. A suspension of particles is passed in front of a light source, usually a Laser beam (Figure 1, page 42). Particles passing in front of the Laser beam scatter light; this is detected by scatter detectors, usually photomultiplier tubes or photodiodes placed in line with the exciting Laser beam (forward scatter) and perpendicular to the Laser beam (side scatter). Forward scatter generally increases with particle size, while side scatter increases with the granularity or optical complexity of the particles. Scatter measurements can therefore be used to determine changes in cell size, shape and optical complexity. As well as scatter detection, the fluorescence properties of particles are also determined using optical filters allowing detection of emitted light of longer wavelength than the excitation Laser. Flow cytometry allows the properties of thousands of particles to be measured per second, generating quantitative data for large numbers of particles within a sample. As well as allowing measurement of particle optical properties, some instruments also allow physical sorting of cells or bacteria (fluorescence-activated cell sorting or FACS).
Although widely used for the analysis of mammalian cells in fields such as immunophenotyping, flow cytometry and FACS also have many applications in the analysis of microbial cells including bacteria and yeast. Although smaller than mammalian cells (bacteria are typically around 1-2µm in size), advances in instrument development mean that microbes can now routinely and simply be analysed and sorted on a single cell basis. The chief advantages of flow cytometry in terms of rapidity, single-cell analysis (so allowing determination of population heterogeneity) and direct observation of cells (so not relying upon growth of cells before analysis) equally apply to microbes and mammalian cells.
How many cells? Monitoring viability and physiology
The simplest use of flow cytometry is counting cells. Many instruments are able to volumetrically count particles (that is, count particles per microlitre); alternatively, ratiometric counting can be used, calibrated against a known number of beads added to samples. Scatter measurements can also be used to detect changes in cell size and shape, for example filamentation of E. coli in response to stress or other morphological changes3 and detection of contaminants in fermentation cultures4.
A major use of flow cytometry for microbial analysis is the determination of viability and physiology. As well as speed (data can be generated in minutes), a major advantage of using flow cytometry for this application is its non-reliance on growth. Many bacteria can exhibit a viable but non-culturable (VBNC) phenotype, meaning that although they are viable, they cannot grow on solid culture medium such as agar plates5, which is a common method of viability assessment for microbial fermentations. This phenotype may be species-specific (some bacteria are unculturable in the laboratory) or induced by stress. The latter situation commonly occurs in recombinant protein production processes, especially those at high biomass concentration. Although these bacteria are able to grow rapidly in a bioreactor, once removed from the bioreactor they are unable to grow on agar plates and so appear to be nonviable. As flow cytometry relies upon direct cellular measurements, it can measure the viability state of each bacterium without the need for growth. When compared to spectrophotometric absorbance measurements (optical density, another common technique for assessing growth of microbial cultures), flow cytometry allows discrimination between live and dead cells.
A wide range of dyes is available for the determination of various aspects of microbial viability and physiology. Viability may be determined in numerous ways, but common approaches rely upon measurement of cell integrity, membrane potential and enzymatic activities. Dyes such as SYTO® 9 (green) and propidium iodide (PI; red) fluoresce strongly when bound to DNA. SYTO 9 is a permeant dye and can pass through cell membranes, but PI is impermeant and cannot enter live bacteria. Dual staining with SYTO 9 and PI (the LIVE/DEAD® BacLIGHTTM system) therefore stains live cells green (Fig. 2a). Dead cells, with a disrupted cell envelope, stain positively with PI, so fluoresce red; PI also reduces green fluorescence through FRET (Förster resonance energy transfer).
Oxonol dyes can be used to measure membrane potential, another important indicator of bacterial viability due to its central role in cellular energy generation. Oxonol dyes such as DiBAC4(3) (Bis-(1,3-Dibutylbarbituric Acid)Trimethine Oxonol) preferentially enter membrane depolarised bacteria, thus allowing detection of ‘injured’ bacteria (Fig. 2b). As it is a green dye, it can be used in conjunction with PI to differentiate between healthy, injured and dead bacteria (Fig. 2c).
Esterase substrates such as Carboxyfluorescein Diacetate (CFDA) permit detection of two aspects of viability. CFDA is non-fluorescent until cleaved by an esterase enzyme present in metabolically active cells, whereupon it becomes fluorescent (Fig. 2d). However, some esterase activity might be present in recently killed cells; the fluorescent carboxyfluorescein moiety rapidly leaves dead cells, leaking through ruptured cell membranes. Therefore, only esterase-positive, intact cells have high fluorescence when stained with CFDA. As with SYTO 9 and DiBAC4(3), CFDA emits green fluorescence and so can be used alongside PI to simultaneously determine two aspects of bacterial physiology. Although many of these dyes can be employed for analysis using a fluorescence microscope, flow cytometry eliminates the need for a trained operator to enumerate cells manually, allows larger numbers of cells to be analysed and generates quantitative data.
These methods of assessing bacterial viability and physiology have been applied to the optimisation of bacterial fermentations generating recombinant proteins. Want et al.6 used PI and DiBAC4(3) to determine physiological changes during fed-batch fermentations of E. coli generating a Fab antibody fragment, an important class of bacterially-synthesised biopharmaceuticals. A similar approach was taken by Hyka et al.7 for Pichia pastoris fermentations using a wider variety of fluorescent dyes. In each case, flow cytometry can quickly reveal subpopulations of dead or injured cells and so allow fermentation scientists to alter process conditions during the fermentation in order to increase viability. Many alternative methods such as determination of viability by colony forming units only generate data after an extended period of time (12-24 hours), so would require a repetition of the fermentation with altered conditions. Flow cytometry can therefore potentially accelerate process development.
How much recombinant protein are the cells generating? Monitoring productivity
A second important factor in optimising a recombinant protein production process is ensuring that the microbial cells are generating the recombinant protein of interest. Fusion of a fluorescent protein such as green fluorescent protein (GFP), originally isolated from the jellyfish Aequorea victoria) to the recombinant protein of interest permits easy measurement using fluorescence as a reporter8. GFP has been optimised for use with flow cytometry9 and so is a widely used tool.
Several studies using a recombinant protein-GFP fusion have shown good correlation between GFP fluorescence intensity and the yield of recombinant protein per cell10,11. In addition, fusion of GFP to the C-terminus of the recombinant protein of interest has also allowed recombinant protein folding to be assessed. Correct folding of the recombinant protein has been seen to result in correct folding of GFP and thus high fluorescence; recombinant protein misfolding (frequently leading to inclusion body formation) leads to lower fluorescence12. Measurement of recombinant protein-GFP fusions using flow cytometry is extremely rapid, especially compared to sodium dodecyl sulfate polyacrylamide gel electrophoresis (SDS-PAGE) or enzyme-linked immunosorbent assay (ELISA) methods for determination of protein concentration. Unlike these more conventional methods, flow cytometry is also able to determine the proportion of bacteria within a population that are productive (GFP+) and so can indicate plasmid loss during recombinant protein production. In addition, measurement of GFP can also be combined with viability determination using propidium iodide to determine the viability of productive and non-productive sub-populations within cultures (Figure 3). These methods can be used to optimise fermentation strategies11 and also other stages of the recombinant protein production process such as culture setup13. As with viability and physiology determination, rapid data acquisition permits identification of non-productive subpopulations of cells during a process and so allows alteration of process conditions in real time.
Of course, a therapeutic protein cannot be administered to a patient with GFP fused to it, and protease cleavage of a recombinant protein adds undesired complexity to the production process, so it is envisaged that recombinant protein-GFP fusions would be used for initial process design and optimisation (from shakeflasks up to bioreactor cultures); for actual production, the non-GFP fused recombinant protein would be generated. An approach avoiding the use of fusion proteins is antibody staining, although this is only usually practical when the recombinant protein is surface-displayed.
Which cells should I use? Selecting and screening hosts
FACS is an established method for selection of the best host cells for recombinant protein production in mammalian systems such as CHO cells; it has recently been applied to selection of microbial hosts as well. Two broad approaches can be taken to screen bacterial producers. First, parallel cultures can be grown (often in 96-well plates or similar) and analysed using flow cytometry. Each culture comprises a different potential host strain, so this approach allows determination of physiology, productivity and heterogeneity of each individual host. Stadlmayr et al.14 used this approach to develop novel promoters for recombinant protein production in the methylotrophic yeast Pichia pastoris.
Alternatively, a mixture of hosts (possibly generated using a random mutagenesis approach) can be grown in a single culture and this mixed population interrogated by flow cytometry; individual microbial cells can subsequently be physically isolated by FACS. This approach has been used to select E. coli host strains that are capable of generating readily-misfolding recombinant proteins in a soluble form15. As described above, GFP fused to the C-terminal of a recombinant protein of interest can be used to determine recombinant protein folding12. Thus, FACS selection of bacteria with high green fluorescence from a culture can be used to isolate strains capable of production of soluble recombinant protein. This selection method could also be performed by selecting green colonies from agar plates; FACS allows not only quantification of green fluorescence but also selection of VBNC bacteria, thus allowing selection from a larger pool of potential modified hosts.
Stadlmayr et al.16 used a similar approach to optimise production of a recombinant antibody fragment in Pichia pastoris by identifying genes that enhance surface display. A library of P. pastoris complementary DNA was expressed in a pool of P. pastoris cells; the ability of this pool of cells to express a surface-displayed antibody fragment, detected using a fluorescently-labelled antibody, was tested. Four successive rounds of FACS were used to select for P. pastoris cells that were better able to generate the recombinant antibody fragment on their surface. This approach could in future be used to select for producer cells with specific abilities such as enhanced recombinant protein folding.
Conclusion
In summary, the three main advantages of flow cytometry (direct observation of cells without the need for growth, assessment of heterogeneity within a population and rapidity of analysis of large numbers of particles) have significant benefits for the optimisation of recombinant proteins in microbial hosts.
References
- Overton TW Recombinant protein production in bacterial hosts. Drug Discov Today. 2014; 19 :590-601.
- Walsh G. Biopharmaceutical benchmarks 2010. Nat Biotechnol. 2010; 28: 917-24.
- Kunze M, Roth S, Gartz E, Büchs J. Pitfalls in optical on-line monitoring for high-throughput screening of microbial systems. Microb Cell Fact. 2014; 13:53.
- Hewitt CJ, Nebe-Von-Caron G. The application of multi-parameter flow cytometry to monitor individual microbial cell physiological state. Adv Biochem Eng Biotechnol. 2004; 89: 197-223.
- Müller S, Nebe-von-Caron G. Functional single-cell analyses: flow cytometry and cell sorting of microbial populations and communities. FEMS Microbiol Rev. 2010; 34: 554-87.
- Want A, Thomas OR, Kara B, Liddell J, Hewitt CJ. (2009) Studies related to antibody fragment (Fab) production in Escherichia coli W3110 fed-batch fermentation processes using multiparameter flow cytometry. Cytometry A. 75: 148-54.
- Hyka P, Züllig T, Ruth C, Looser V, Meier C, Klein J, Melzoch K, Meyer HP, Glieder A, Kovar K. Combined use of fluorescent dyes and flow cytometry to quantify the physiological state of Pichia pastoris during the production of heterologous proteins in high-cell-density fed-batch cultures. Appl Environ Microbiol. 2010; 76: 4486-96.
- Vizcaino-Caston I, Wyre C, Overton TW. Fluorescent proteins in microbial biotechnology -new proteins and new applications. Biotechnol Lett. 2012; 34: 175-86.
- Cormack BP, Valdivia RH, Falkow S. FACS-optimized mutants of the green fluorescent protein (GFP). Gene. 1996; 173: 33-8.
- Jones JJ, Bridges AM, Fosberry AP, Gardner S, Lowers RR, Newby RR, James PJ, Hall RM & Jenkins O Potential of real-time measurement of GFP-fusion proteins. J Biotechnol 109: 2004; 201–211.
- Sevastsyanovich Y, Alfasi S, Overton T, Hall R, Jones J, Hewitt C, Cole J. Exploitation of GFP fusion proteins and stress avoidance as a generic strategy for the production of high-quality recombinant proteins. FEMS Microbiol Lett. 2009; 299: 86-94.
- Waldo GS, Standish BM, Berendzen J & Terwilliger TC Rapid protein-folding assay using green fluorescent protein. Nat Biotechnol. 1999; 17: 691–695.
- Wyre C, Overton TW. Flow cytometric analysis of E. coli on agar plates: implications for recombinant protein production. Biotechnol Lett. 2014; 36: 1485-94.
- Stadlmayr G, Mecklenbräuker A, Rothmüller M, Maurer M, Sauer M, Mattanovich D, Gasser B. Identification and characterisation of novel Pichia pastoris promoters for heterologous protein production. J Biotechnol. 2010; 150: 519-29.
- Alfasi S, Sevastsyanovich Y, Zaffaroni L, Griffiths L, Hall R, Cole J. Use of GFP fusions for the isolation of Escherichia coli strains for improved production of different target recombinant proteins. J Biotechnol. 2011; 156: 11-21.
- Stadlmayr G, Benakovitsch K, Gasser B, Mattanovich D, Sauer M. Genome-scale analysis of library sorting (GALibSo): Isolation of secretion enhancing factors for recombinant protein production in Pichia pastoris. Biotechnol Bioeng. 2010; 105: 543-55.
Biography
Tim Overton is a lecturer in Bioengineering at the University of Birmingham. He is a biochemist and molecular microbiologist by training and his current research focuses on the development, optimisation and intensification of microbial bioprocesses including recombinant protein production, biopolymer generation and biotransformations. He uses flow cytometry and FACS as essential tools for bioprocess development. He also collaborates with microbiologists to apply flow cytometry and FACS in order to answer fundamental questions about bacterial physiology and behaviour.