Improved in vitro renal tubular drug transport modelling to meet regulatory and development cost challenges
Posted: 15 December 2020 | Dr Colin Brown (Newcells Biotech) | No comments yet
Due to the high costs associated with drug discovery and the clinical demand for effective drugs, in vitro human cell-based kidney models using renal proximal tubule epithelia are becoming popular tools for early-stage testing. It is increasingly more important that in vitro models of renal drug transport are physiologically representative in order to accurately model nephrotoxicity and drug‑to‑drug interactions and predict or avoid incidences of drug-induced kidney injury in the later stages of development. In this article, Dr Colin Brown, director of ADMET technology at Newcells Biotech, discusses this further and considers future modelling options to address these challenges.
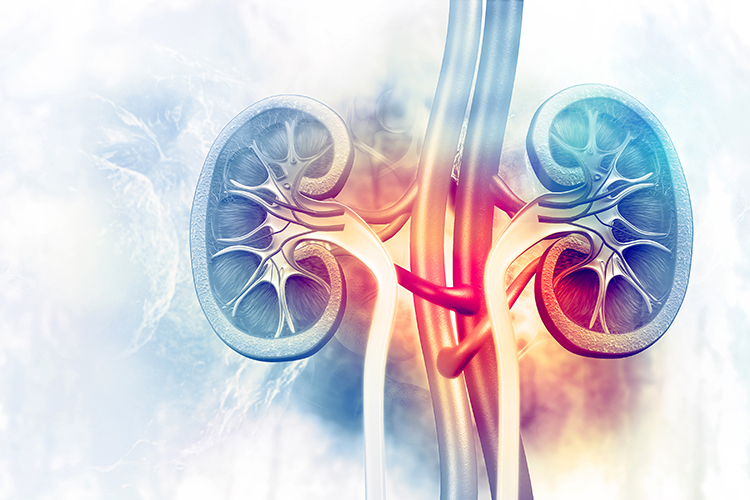
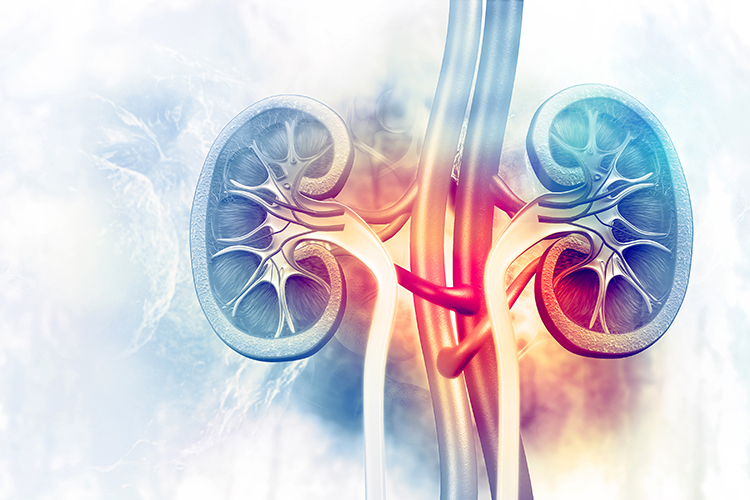
ONE-THIRD OF all drugs and drug candidates are cleared renally.1 The kidney’s important role in drug clearance makes it prone to damage; drug-induced kidney injury (DIKI) is estimated to be responsible for up to 60 percent of all acute kidney injury (AKI) cases, while 10 percent of post‑market drug attrition is due to DIKI.2-4 It is estimated that drugs are responsible for approximately 20 percent of acute renal failure episodes and this figure increases dramatically in the elderly where the incidence of drug-induced nephrotoxicity is reported to be as high as 66 percent.5-8
The renal proximal tubule is the major site of drug transport due to the concerted action of various uptake and efflux mechanisms. Costs of drug development have been increasing since the 1970s to $2.6 billion today.9 This underlines the importance of reliable, predictive and cost-efficient in vitro models for use in development and supporting regulatory submission.
Higher uptake of a drug into proximal tubule epithelial cells (PTCs) than efflux results in intracellular accumulation of drugs and may result in toxicity. Furthermore, drug-drug interactions (DDIs) can interfere with drug transport mechanisms and are a huge economic burden on healthcare that is expected to worsen with an ageing population and increasing polypharmacy. DDI most frequently occurs due to interactions with drug metabolising enzymes or drug transporters. Traditional renal in vitro assays often are not sufficient to accurately identify DDIs and nephrotoxicity, while pre-clinical animal models are not predictive due to species differences10 – these factors present a significant roadblock to the efficient development of safe drugs.
Traditional renal in vitro assays often are not sufficient to accurately identify DDIs and nephrotoxicity, while pre‑clinical animal models are not predictive due to species differences”
In drug development, existing knowledge of drug distribution routes and possible DDIs is essential for the downstream success of clinic-bound compounds. US Food and Drug Administration (FDA) guidelines, as of 2020, recommend certain in vitro investigational steps concerning a compound’s potential interaction with drug transporters if ADME (absorption, distribution, metabolism and excretion) data suggest that active secretion of the parent drug is greater than or equal to 25 percent of total clearance.11 They define the key transporters as apical-spanning efflux pumps P-glycoprotein (P-gp; ABCB1), breast cancer resistance protein (BCRP; ABCG2) and multidrug and toxin extrusion transporters 1/2 (MATE1/2k; SLC47A1/2), as well as organic anion transporters 1/3 (OAT1/3; SLC22A6/8) and organic cation 2 (OCT2; SLC22A2) expressed on the basolateral membrane of proximal tubular cells. Therefore, to produce relevant and useful data for regulatory submissions, it is crucial to choose your pre-clinical models with care.
The current renal models
Traditionally, animal and human cell lines such as human (HEK293), pig kidney-derived (LLC-PK1) and Madin-Darby canine kidney (MDCK) cell lines12-16 have been used to model transporter-mediated uptake and to study movement of candidate drug molecules within the kidney. Cells that form polarised monolayers and tight junctions can also be double-transfected, allowing multi‑compartmental evaluation of a compound’s transport.13 Though these models are useful for determining uptake kinetics and possible DDIs of new compounds, they only express two transporters at most and therefore do not offer a holistic view of the handling of a compound.
Human-derived cells, such as the immortalised HK-2 cell line, edged the field closer towards a more in vivo model, although they lacked key features and transporters of in vivo tubules.17 They fall short of true in vivo features to model human clinical relevance, with reported issues that include: tight barrier formation; cells that lack the full complement of transporters in the correct ratios; and the use of animal cells as transporter vectors.18-21 Using cell models derived from animals can also introduce various discrepancies in transporter expression. For instance, the rodent proximal tubule contains OCT1 at the basolateral membrane, while apical expression of MATE2-K has not been detected.22 Recently, differential protein expression in the proximal tubule between species has been highlighted,13,23,24 suggesting probable differences in drug handling for a wide range of substrates, which can be problematic when transferring data from pre-clinical species.
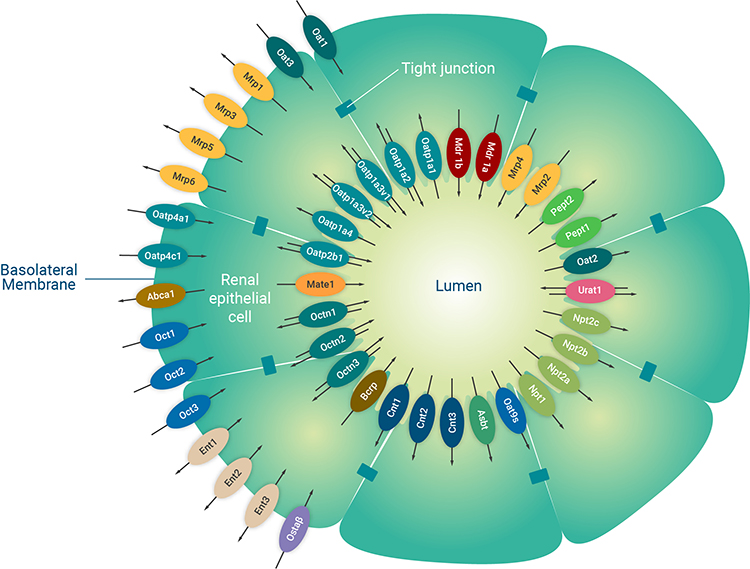
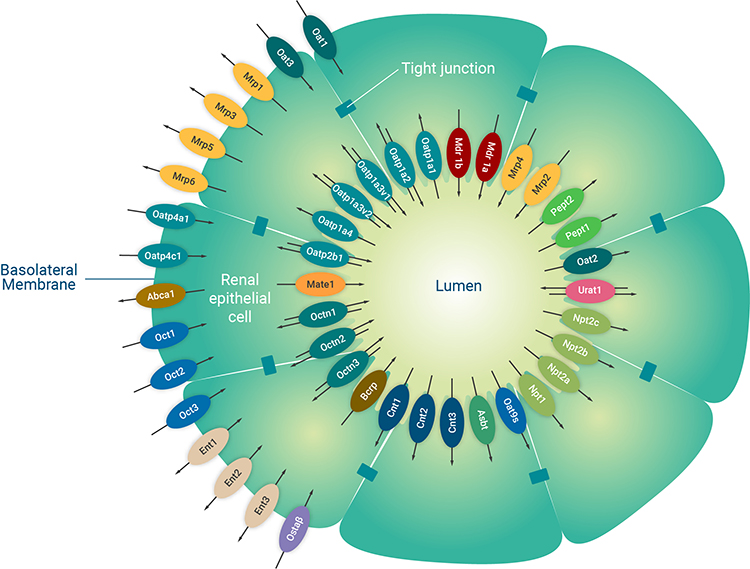
Figure 1: A schematic diagram of the major renal proximal tubule epithelial cell transporters. The renal proximal tubule is a specialised polarised cell layer in the kidney that is a major site of drug secretion and absorption mediated by membrane-located transporters. Image used and adapted with permission from SOLVO Biotechnology, a Charles River Company.
To date, the most predictive and representative of in vivo results has been to culture primary cells sourced directly from fresh tissue that retain a higher abundance of functional transporters. In vitro human cell-based kidney models using renal proximal tubule epithelial cells are now becoming popular for early-stage testing of nephrotoxicity.
Functional activity of many of the key transporters has been determined in primary cultures with substrates, including Para-aminohippurate (PAH) and creatinine.25,26 More recently, a transporter-dependant model of nephrotoxicity has demonstrated functionality across a wide range of key transporters, allowing a high rate of predictivity for nephrotoxins, including test compounds that had been through traditional pre-clinical models.27 Data has also been generated to demonstrate the consistency of cisplatin-induced toxicity over multiple donors and the reproducibility of data generated from primary cells.
The same model has more recently shown species-species differences in the handling of a commercial herbicide, again demonstrating a likely function of OATs in the model28 and exposing the limitations of animal models for transport studies. Criticisms of primary cultures have been the longevity of cultures and the requirement to maintain a consistent supply of fresh human tissue. These aside, freshly isolated proximal tubule cells arguably remain the gold standard of both trans‑epithelial flux and nephrotoxicity prediction assays.
Future modelling options
Generating a model of drug transport within the proximal tubule from stem cells has gained momentum in recent years; theoretically yielding an infinite population of proximal tubule cells with relevant transporters. A challenge is the maturity of the cells after their differentiation, with transcriptomic maturity resembling cells in the first-trimester kidney being reported.29 There have been attempts to increase the maturity of kidney organoids, while also reducing their time in culture; however, transporter expression levels and function have not yet been investigated.30 An interesting recent development has seen the collection of adult stem cells from urine samples, which require less complex medium components and culture time when compared with directing the differentiation of iPSCs.31 Currently, however, the stem-cell-derived models lack the extensive functional expression of transporters validation data of primary cell culture models. When this hurdle is overcome, the application of stem-cell-derived models holds great promise for the investigation of renal drug transport.
In vitro human cell-based kidney models using renal proximal tubule epithelial cells are now becoming popular for early-stage testing of nephrotoxicity”
To mimic the aspects of the PTC microenvironment, in vitro culture platforms can range from two-dimensional (2D) to advanced models that recreate the cells’ microenvironment. Culture of PTCs on inserts creates a bi‑compartmental model and improved formation of a PTC monolayer.32 The increasing complexity of three-dimensional (3D) models could limit throughput in renal drug transport studies; most published models contain one tubule per device and use pumps to induce fluid shear stress (FSS)19,33-36 although more chips could be combined on one device to allow medium-throughput testing of multiple compounds (Vriend, et al, unpublished).
Focus has shifted towards transferability of advanced renal in vitro models of nephrotoxicity and drug transport in microphysiological systems using primary human PTCs. These systems have demonstrated a more physiological response and function when compared to immortalised PTCs.37 Tubular absorption was successfully predicted by in vitro to in vivo extrapolation (IVIVE)38 and transport kinetics in chronic kidney disease were accurately predicted by IVIVE translation of active secretion of indoxyl sulfate in a hollow fibre membrane.39 The marked increase in the development of and transferability of advanced renal in vitro 3D models in recent years is promising, but no model has yet provided sufficient validation data. Furthermore, advanced renal in vitro models are often not compatible with conventional lab equipment, are low throughput and are not proven to be cost efficient. However, these recent advances are promising and could pave the way for a new wave of improved models to study renal drug transport.
As the requirement for in vivo pre-clinical testing is driven by regulatory and cost consideration we have multiple cell and culture models available for renal drug transport and an improved understanding of renal drug transport. It becomes clear that various types of complexity can be added to mimic PTC drug transport in vitro and we should allow the research question to determine the level of complexity required and establish which in vitro model is fit for purpose. For instance, profiling of renal clearance of a single compound requires a model of higher in vivo resemblance, preferably in primary PTCs. On the other hand, possible DDIs are best studied in more simplified models, such as transfected cell lines. Improving the predictability of renal drug transport and DDIs is not limited by using advanced in vitro models but could be extended by combining IVIVE and physiologically based pharmacokinetic (PBPK) modelling.
About the author
Dr Colin Brown is a leading expert in kidney transport, with research interests in renal, hepatic and GI drug transporters. He has developed and commercialised, through Newcells Biotech, a primary cell-based assay for measuring kidney transport and toxicity. The assay has been used in a range of study protocols globally with pharma and other industries to investigate drug transport, drug-drug interaction and nephrotoxicity.
Declaration of competing interest
The authors declare the following financial interests/ personal relationships, which may be considered as potential competing interests: All authors are employees of Newcells Biotech Limited.
References
- Morrissey KM, Stocker SL, Wittwer MB, Xu L, Giacomini KM: Renal transporters in drug development. Annu Rev Pharmacol Toxicol (2013) 53:503-529.
- Cook D, Brown D, Alexander R, March R, Morgan P, Satterthwaite G, Pangalos MN: Lessons learned from the fate of Astrazeneca’s drug pipeline: A five-dimensional framework. Nat Rev Drug Discov (2014) 13(6):419-431.
- Ghane Shahrbaf F, Assadi F: Drug-induced renal disorders. J Renal Inj Prev (2015) 4(3):57-60.
- Awdishu L, Mehta RL: The 6r’s of drug induced nephrotoxicity. BMC Nephrol (2017) 18(1):124.
- Kaufman J, Dhakal M, Patel B, Hamburger R: Community-acquired acute renal failure. Am J Kidney Dis (1991) 17(2):191-198.
- Nash K, Hafeez A, Hou S: Hospital-acquired renal insufficiency. Am J Kidney Dis (2002) 39(5): 930-936.
- Bellomo R: The epidemiology of acute renal failure:1975 versus 2005. Curr Opin Crit Care (2006) 12(6): 557-560.
- Kohli HS, Bhaskaran MC, Muthukumar T, Thennarasu K, Sud K, Jha V, Gupta KL, Sakhuja V: Treatment-related acute renal failure in the elderly: a hospital-based prospective study. Nephrol Dial Transplant (2000) 15(2):212-217.
- Paul SM, Mytelka DS, Dunwiddie CT, Persinger CC, Munos BH, Lindborg SR, Schacht AL: How to improve r&d productivity: The pharmaceutical industry’s grand challenge. Nat Rev Drug Discov (2010) 9(3):203-214.
- Magee TV, Brown MF, Starr JT, Ackley DC, Abramite JA, Aubrecht J, Butler A, Crandon JL, Dib-Hajj F, Flanagan ME, Granskog K, et al: Discovery of dap-3 polymyxin analogues for the treatment of multidrug-resistant gram-negative nosocomial infections. J Med Chem (2013) 56(12):5079-5093.
- Food and Drug Administration (FDA): In vitro drug interaction studies — cytochrome p450 enzyme- and transporter-mediated drug interactions guidance for industry: (2020). https://www.fda.gov/regulatory-information/search-fda-guidance-documents/vitro-drug-interaction-studies-cytochrome-p450-enzyme-and-transporter-mediated-drug-interactions
- Parvez MM, Shin HJ, Jung JA, Shin JG: Evaluation of para-aminosalicylic acid as a substrate of multiple solute carrier uptake transporters and possible drug interactions with nonsteroidal anti-inflammatory drugs in vitro. Antimicrob Agents Chemother (2017) 61(5).
- Muller F, Weitz D, Derdau V, Sandvoss M, Mertsch K, Konig J, Fromm MF: Contribution of mate1 to renal secretion of the nmda receptor antagonist memantine. Mol Pharm (2017) 14(9):2991-2998.
- Hotchkiss AG, Berrigan L, Pelis RM: Organic anion transporter 2 transcript variant 1 shows broad ligand selectivity when expressed in multiple cell lines. Front Pharmacol (2015) 6(216.
- Li J, Wang Y, Hidalgo IJ: Kinetic analysis of human and canine p-glycoprotein-mediated drug transport in mdr1-mdck cell model: Approaches to reduce false-negative substrate classification. J Pharm Sci (2013) 102(9):3436-3446.
- Tahara K, Kagawa Y, Takaai M, Taguchi M, Hashimoto Y: Directional transcellular transport of bisoprolol in p-glycoprotein-expressed llc-ga5-col150 cells, but not in renal epithelial llc-pk1 cells. Drug Metab Pharmacokinet (2008) 23(5):340-346.
- Jenkinson SE, Chung GW, van Loon E, Bakar NS, Dalzell AM, Brown CD: The limitations of renal epithelial cell line hk-2 as a model of drug transporter expression and function in the proximal tubule. Pflugers Arch (2012) 464(6):601-611.
- Wilmer MJ, Saleem MA, Masereeuw R, Ni L, van der Velden TJ, Russel FG, Mathieson PW, Monnens LA, van den Heuvel LP, Levtchenko EN: Novel conditionally immortalized human proximal tubule cell line expressing functional influx and efflux transporters. Cell Tissue Res (2010) 339(2):449-457.
- Jansen J, De Napoli IE, Fedecostante M, Schophuizen CM, Chevtchik NV, Wilmer MJ, van Asbeck AH, Croes HJ, Pertijs JC, Wetzels JF, Hilbrands LB, et al: Human proximal tubule epithelial cells cultured on hollow fibers: Living membranes that actively transport organic cations. Sci Rep (2015) 5(16702.
- Jansen J, Schophuizen CM, Wilmer MJ, Lahham SH, Mutsaers HA, Wetzels JF, Bank RA, van den Heuvel LP, Hoenderop JG, Masereeuw R: A morphological and functional comparison of proximal tubule cell lines established from human urine and kidney tissue. Exp Cell Res (2014) 323(1):87-99.
- Secker PF, Schlichenmaier N, Beilmann M, Deschl U, Dietrich DR: Functional transepithelial transport measurements to detect nephrotoxicity in vitro using the rptec/tert1 cell line. Arch Toxicol (2019) 93(7):1965-1978.
- Nieskens TT, Peters JG, Schreurs MJ, Smits N, Woestenenk R, Jansen K, van der Made TK, Roring M, Hilgendorf C, Wilmer MJ, Masereeuw R: A human renal proximal tubule cell line with stable organic anion transporter 1 and 3 expression predictive for antiviral-induced toxicity. AAPS J (2016) 18(2):465-475.
- Nieskens TTG, Peters JGP, Dabaghie D, Korte D, Jansen K, Van Asbeck AH, Tavraz NN, Friedrich T, Russel FGM, Masereeuw R, Wilmer MJ: Expression of organic anion transporter 1 or 3 in human kidney proximal tubule cells reduces cisplatin sensitivity. Drug Metab Dispos (2018) 46(5):592-599.
- Kuteykin-Teplyakov K, Luna-Tortos C, Ambroziak K, Loscher W: Differences in the expression of endogenous efflux transporters in mdr1-transfected versus wildtype cell lines affect p-glycoprotein mediated drug transport. Br J Pharmacol (2010) 160(6):1453-1463.
- Brown CD, Sayer R, Windass AS, Haslam IS, De Broe ME, D’Haese PC, Verhulst A: Characterisation of human tubular cell monolayers as a model of proximal tubular xenobiotic handling. Toxicol Appl Pharmacol (2008) 233(3):428-438.
- Sanchez-Romero N, Martinez-Gimeno L, Caetano-Pinto P, Saez B, Sanchez-Zalabardo JM, Masereeuw R, Gimenez I: A simple method for the isolation and detailed characterization of primary human proximal tubule cells for renal replacement therapy. Int J Artif Organs (2020) 43(1):45-57.
- Bajaj P, Chung G, Pye K, Yukawa T, Imanishi A, Takai Y, Brown C, Wagoner MP: Freshly isolated primary human proximal tubule cells as an in vitro model for the detection of renal tubular toxicity. Toxicology (2020) 442(152535.
- Bartels M, Brown C, Chung G, Chan M, Terry C, Gehen S, Corvaro M: Review of the pharmacokinetics and metabolism of triclopyr herbicide in mammals: Impact on safety assessments. Regul Toxicol Pharmacol (2020) 116(104714.
- Takasato M, Er PX, Chiu HS, Maier B, Baillie GJ, Ferguson C, Parton RG, Wolvetang EJ, Roost MS, Chuva de Sousa Lopes SM, Little MH: Kidney organoids from human ips cells contain multiple lineages and model human nephrogenesis. Nature (2015) 526(7574):564-568.
- Garreta E, Prado P, Tarantino C, Oria R, Fanlo L, Marti E, Zalvidea D, Trepat X, Roca-Cusachs P, Gavalda-Navarro A, Cozzuto L, et al: Fine tuning the extracellular environment accelerates the derivation of kidney organoids from human pluripotent stem cells. Nat Mater (2019) 18(4):397-405.
- Schutgens F, Rookmaaker MB, Margaritis T, Rios A, Ammerlaan C, Jansen J, Gijzen L, Vormann M, Vonk A, Viveen M, Yengej FY, et al: Tubuloids derived from human adult kidney and urine for personalized disease modeling. Nat Biotechnol (2019) 37(3):303-313.
- Orosz DE, Woost PG, Kolb RJ, Finesilver MB, Jin W, Frisa PS, Choo CK, Yau CF, Chan KW, Resnick MI, Douglas JG, et al: Growth, immortalization, and differentiation potential of normal adult human proximal tubule cells. In Vitro Cell Dev Biol Anim (2004) 40(1-2):22-34.
- Jang KJ, Mehr AP, Hamilton GA, McPartlin LA, Chung S, Suh KY, Ingber DE: Human kidney proximal tubule-on-a-chip for drug transport and nephrotoxicity assessment. Integr Biol (Camb) (2013) 5(9):1119-1129.
- Homan KA, Kolesky DB, Skylar-Scott MA, Herrmann J, Obuobi H, Moisan A, Lewis JA: Bioprinting of 3d convoluted renal proximal tubules on perfusable chips. Sci Rep (2016) 6(34845).
- Jansen J, Fedecostante M, Wilmer MJ, Peters JG, Kreuser UM, van den Broek PH, Mensink RA, Boltje TJ, Stamatialis D, Wetzels JF, van den Heuvel LP, et al: Bioengineered kidney tubules efficiently excrete uremic toxins. Sci Rep (2016) 6(26715.
- Weber EJ, Chapron A, Chapron BD, Voellinger JL, Lidberg KA, Yeung CK, Wang Z, Yamaura Y, Hailey DW, Neumann T, Shen DD et al: Development of a microphysiological model of human kidney proximal tubule function. Kidney Int (2016) 90(3):627-637.
- Sakolish C, Weber EJ, Kelly EJ, Himmelfarb J, Mouneimne R, Grimm FA, House JS, Wade T, Han A, Chiu WA, Rusyn I: Technology transfer of the microphysiological systems: A case study of the human proximal tubule tissue chip. Sci Rep (2018) 8(1):14882.
- Sakolish C, Chen Z, Dalaijamts C, Mitra K, Liu Y, Fulton T, Wade TL, Kelly EJ, Rusyn I, Chiu WA: Predicting tubular reabsorption with a human kidney proximal tubule tissue-on-a-chip and physiologically-based modeling. Toxicol In Vitro (2020) 63(104752.
- van der Made TK, Fedecostante M, Scotcher D, Rostami-Hodjegan A, Sastre Torano J, Middel I, Koster AS, Gerritsen KG, Jankowski V, Jankowski J, Hoenderop JGJ, et al: Quantitative translation of microfluidic transporter in vitro data to in vivo reveals impaired albumin-facilitated indoxyl sulfate secretion in chronic kidney disease. Mol Pharm (2019) 16(11):4551-4562.
Issue
Related topics
Assays, Drug Development, Drug Safety, Preclinical Research, Toxicology
Related organisations
Related diseases & conditions
Acute kidney injury (AKI), Drug-induced kidney injury (DIKI)